The atomic force microscope is a sophisticated instrument which helps delineate the characteristics of polymers by revealing physical properties and mechanical behavior at nanoscale, as well as providing images. This article discusses the advanced features and benefits of Asylum Research CypherTM and MFP-3DTM AFMs in this field.
Polymers are useful in a host of applications. Their properties can be tuned and may be customized to suit different uses. Moreover, they are often less costly, sturdier and more environmentally friendly than other materials. In order to produce and bring into use a new polymer, the structure, processing, characteristics and performance must all be understood. This is achievable at micro- or molecular level if the data acquired is of this resolution.
Figure 1 shows AFM usage in analyzing the basic principles underlying polymers, while Figure 2 shows its employment in engineering a particular solution of a polymer. The point is that AFM plays a vital role in assessing polymers at these small length scales [1-4]. The high spatial resolution brings polymer morphology into view at resolutions below micrometers or even nanometers. However, much more data can be obtained using AFMs, such as molecular forces, mechanical, thermal and electrical mapping, and finding the effects of heat or solvents in realistic situations.
.jpg)
Figure 1: Morphology of PS-PEP diblock copolymer film – Tapping mode phase image of a polystyrene-block-poly(ethylenealt-propylene) (PS-PEP) film on a silicon wafer. The darker region on the left side with lower phase corresponds to the high side of a 16 nm step in the wafer. In this region and the darker curved region on the right, the film forms a PS wetting layer. In the lighter region with higher phase, the film contains a single layer of spherical PEP microdomains. Several vertical rows of aligned microdomains are seen on the low side of the step. Diblock copolymers can self assemble into complex periodic structures, a property that has attracted interest for their use as templates in nanolithography. Understanding how film thickness and step height affect domain location and orientation aids in developing block copolymer templates. Imaged with the MFP-3D AFM; scan width 2 μm, Z (phase) scale 10°. Adapted from Ref. 5.
.jpg)
Figure 2: Distribution of components in EVA-EPP-carbon black blends – Images of tapping mode topography (left) and phase (right) of semiconducting polymer blends. The blends contained two polyolefin copolymers, poly(ethylene vinyl acetate) (EVA) and ethylene-propylene copolymer (EPP), mixed with carbon black (CB). The images correspond to blends with 10 wt % (top) and 30 wt % (bottom) EPP. Carbon black is an economical filler used in many practical applications to create electrically conducting polymers. However, addition of CB can significantly affect blend qualities such as viscosity. The use of two copolymers achieved a balance between performance (electrical volume resistivity) and processing (melt flow rate). The phase images clearly differentiate individual blend components, allowing the effect of component distribution on conductivity to be studied. Imaged with the MFP-3D AFM, scan size 20 μm. For more information see Ref. 6.
Investigating Morphology and Structure
Polymer structures change at different scales of length. These smaller scale structures include a brush configuration, and if single molecules are visualized, chain packing; for crystalline polymers, lamellar formations; and with block or graft copolymers, microphase separation. Features visible on larger scales include pores or fillers, and for blends of polymers, interfacial phases. Effects of processing like etching, heat or strain are also visible on the surface roughness or finish. The study of nanoparticles, nanofibers and nanodevices made of polymer includes statistical analysis of both individual components and the whole assembly’s shape and size.
The AFM allows the structure to be visualized via nanoscale topography of the surface, typically in tapping mode. This mode yields images with excellent spatial resolution, up to molecular and atomic structure, as Figure 3 reveals. The small area in contact between the tip and the sample is responsible for the high resolution. This in turn is achieved by using ultrasharp tipped probes, in addition to the tapping mode which results in very gentle sideways and vertical forces between the tip and the sample.
As Figure 4 shows, AFM imaging has recently become much faster with the emergence of newer AFMs, such as the Cypher AFMs from Asylum Research. This is due to the use of small cantilevers. Not only do smaller cantilevers have higher resonant frequencies, they are also able to provide improved resolution and control for very small forces in the subpiconewton range, which is extremely crucial when investigating polymers which are delicate and easily undergo deformation.
.jpg)
Figure 3: Molecular and crystalline structure of rubrene – Tapping mode topography images of crystalline rubrene imaged with the Cypher S AFM. Rubrene is a polycyclic aromatic hydrocarbon used as an organic semiconductor in organic light emitting diodes. The main color image with scan size 5 μm shows single steps of the crystalline lattice. The inset black-and-white image reveals molecular-level structure, highlighting the superb spatial resolution of Cypher AFMs, even in air. Scan size 20 nm. Sample courtesy of Rutgers University.
.jpg)
Figure 4: PHB/V spherulite crystallization – Tapping mode phase images of a polyhydroxybutyrate-co-valerate (PHB/V) spherulite crystallizing at room temperature over a period of three minutes. Scan size 1.5 μm. The scan rate of 40 Hz, or approximately 10 seconds per frame, allows clear visualization of the crystallization process. Imaged with the Cypher AFM. Sample courtesy of the University of Sheffield.
Using Asylum AFMs for Topographic Imaging
- All Asylum AFMs use closed-loop scanning which has the advantage of repeatable and precise scanning motions due to the use of position sensors. As a result, image distortions are avoided, with very precise offsets, while specific scan areas are zoomed in to. The Cypher 5 and ES, as well as the MFP-3D InfinityTM all have the most advanced sensors with remarkably low noise, down to 35 pm and 60 pm in the Z, and X and Y, axes respectively.
- The Cypher family AFMs use small cantilevers of less than 10 microns length, so that imaging goes faster, and standard line scan rates are up to 10-40 Hz. These also have better resolution and lower noise, and thus the DNA helix and single point atomic defects are now imaged on a routine basis.
- GetStartedTM is integrated with the MFP-3D Infinity and all Cypher AFMs to automate image parameters. Even before the first tip-sample contact, the parameters are defined automatically using a predictive algorithm. This protects both sample and tip against wear due to non-optimal contact, and also ensures the acquisition of excellent data starting from scan line 1.
|
Measuring Forces and Deformation
The cantilever used in AFMs is one which is built with extreme force sensitivity, which makes it ideal for the measurement of a range of force magnitudes from piconewtons to micronewtons. This contains most polymer interactions too. There are two common ways to measure force: either single molecules are pulled to analyze the forces at molecular level, or polymeric materials are indented to evaluate their elastic and viscoelastic return following deformation.
When the single molecule approach is used, one molecule is stretched between the tip of the AFM and the sample surface, and the deflection of the cantilever is measured. Once the spring constant and deflection sensitivity of the cantilever is known, a force vs. distance plot is generated. These force curves provide information about intramolecular forces, like the elasticity of the single chain, or conformational transitions, as well as intermolecular forces, including interactions between polymer and solvent, and surface adhesion and desorption.
These forces thus act inside or between the molecules. One case of force spectroscopy measurement is shown in Figure 5, using poly-L-lysine molecules during their desorption from a hydrophobic surface.
The other method uses measurements of the deflection produced in the cantilever as the AFM tip indents the sample. Conventionally used cantilevers apply a force that is high enough to cause deformation in the polymers which have modulus ranging from kPa to a few GPa. When a model is used to examine the indentation of the sample by the tip, data is generated on the elastic and viscoelastic characteristics of the sample at nanoscale.
Asylum AFMs for Measuring Force
- GetRealTM is integrated into all Asylum AFMs, being a software to calibrate the cantilever spring constant and deflection sensitivity automatically at one click, without having to touch the surface of the sample.
- All Asylum AFMs have force measurements which are thermally limited and use Z sensors for low noise. This allows measurement of both axes of the force curves with as much sensitivity and accuracy as possible.
- All Asylum AFMs are programmed to analyze force curves with sophisticated features. Several pre-set indentation models are available, such as Hertz/Sneddon, Johnson-Kendall-Roberts (JKR), Derjaguin-Müller-Toporov (DMT), and Oliver-Pharr. In addition there is a guide to model selection which shows the plasticity index, the ratio of force to adhesion, and Tabor coefficient calculation.
|
Mapping Nanomechanical Properties
Polymers must have certain mechanical attributes to suit their varied applications, such as food packaging to flexible electronic devices. Sometimes one or more components with phase separation, or a filler, could be added to enhance the mechanical performance. In most cases they are at nanoscale and thus their mechanical properties must be measured at this spatial resolution.
Asylum has multiple techniques for nanomechanical studies, from simple methods of quantitation to advanced quantitative techniques. In many situations the two are used to complement each other in a way that informs the observer about the polymer sample.
The introduction of the tapping mode for phase imaging created much interest in the late nineties. This has led to the emergence of phase imaging as a very useful tool for characterizing polymers, since it can resolve the fine structure of the molecule and distinguish various components of the material.
Sometimes interpretation of the results becomes difficult because of the influence of the mode of storage and dissipation of elastic energy and viscous energy by the material, respectively, (the loss tangent) in addition to other forces that cause dissipation. Yet phase imaging is still a relatively easy and widespread method of acquiring qualitative data on material properties.
Another way to obtain a qualitative map of material property changes is the use of bimodal imaging or Dual ACTM which is similar to the tapping mode with phase imaging at bottom, with the difference being the driving of an additional cantilever mode along with the first mode. Topography and phase measurements from the first mode are taken along with amplitude and phase response measurements from the second mode. Again, the interpretation is sometimes complicated, but its use remains valid for acquiring contrast where this is not possible by phase imaging.
The Asylum NanomechProTM Toolkit lists many methods, among which the unique AM-FM Viscoelastic Mapping Mode is ideal for polymers. Like bimodal imaging, this technique makes use of tapping mode to operate at two cantilever mode frequencies at the same time. The second mode is tracked for its frequency and correlated with the stiffness of the sample.
The first mode is related to the loss tangent of the sample through its phase and amplitude. The net result is clear quantitative mapping of the elastic storage modulus and the viscoelastic loss modulus, or loss tangent, but with the rapidity expected with the tapping mode and with sample preservation. The large range of applications possible with the AM-FM mode, making it possible to test almost any polymer, is because of the impressive breadth of its measurement range from under 1 MPa to over 100 GPa. One instance where AM-FM mode is used to map a polymer assembled from multiple components is shown in Figure 6.
.jpg)
Figure 5: Single molecule forces for poly-L-lysine homopolymer – (top) Graphic of force spectroscopy concepts. A single polymer molecule is covalently attached to the AFM tip by a linker (here, polyethylene glycol, PEG). (bottom) Force-distance curve for poly-L-lysine on a hydrophobic self-assembled monolayer (SAM) in water. The force plateau indicates the desorption of a single polymer from the surface. Histograms obtained from multiple force curves provide the average desorption force (inset, top) and detachment length (inset, bottom) Acquired with the MFP-3D AFM. Adapted from Ref. 7.
.jpg)
Figure 6: Mechanical mapping of a bonded polymer interface – AM-FM Viscoelastic Mapping Mode images and histograms of (left) loss tangent and (right) second mode frequency overlaid on topography for a rubber-epoxy-latex sandwich. Imaged with the Cypher S AFM; scan size 5 μm. Different sample components are clearly distinguished by the AM loss tangent of viscoelastic damping. They are also resolved by the FM frequency, which is proportional to elastic stiffness, despite very similar modulus values for latex (~40 MPa) and rubber (~43 MPa).
.jpg)
Figure 7: Modulus mapping of PS-PCL blend – Elastic modulus overlaid on topography for a polystyrene (PS)-polycaprolactone (PCL) blend. Imaged with Fast Force Mapping Mode on the MFP-3D Infinity AFM; scan size 4 μm. The 1024x1024 image size would be impractically slow to obtain with conventional force volume techniques. It provides superb lateral resolution, resolving features as small as approximately 10 nm. As expected from bulk literature values, PS regions (yellow)have higher modulus (approximately 3 GPa) than PCL regions (purple, approximately 350 MPa). The biodegradable nature of polycaprolactone is valuable in development of new bioblend materials.
Force curves are one established method of elastic modulus measurement. It is suitable for when point measurements are in view, but mapping using this technique is so slow as to be non-feasible. Typically, a 256x256 pixel array is acquired in more than 18 hours, at 1 second per pixel.
This issue is resolved by the Fast Force Mapping Mode from Asylum, integrated into the MFP-3D Infinity AFM, which has force curve acquisition rates of up to 300 Hz, thus taking below 10 minutes to acquire a 256x256 pixel image of complete deflection vs Z sensor force curves. At this speed there are still no curves found missing nor is there any invisible manipulation of the data. Figure 7 shows how Fast Force Mapping is carried out on a phase-separated polymer blend.
Another special method pioneered by Asylum is the Contact Resonance Viscoelastic Mapping Mode, used to measure elastic storage modulus as well as viscoelastic loss modulus on polymers with relatively high stiffness, modulus of 1 GPa or more.
This mode takes advantage of the high sensitivity of the cantilever resonance to any small variations in the mechanical characteristics of the sample after bringing the tip in contact. In common with all these above-mentioned techniques, Contact Resonance can be used either for rapid qualitative mapping, employing the least possible calibration, or for accurate quantitative results after calibrating against a material whose properties are known.
Asylum AFMs for Mapping Nanomechanical Properties
- Many techniques are described in the Asylum NanomechPro Toolkit in order to help select the best technique for each application, thus comparing the results obtained using several different techniques.
- Both AM-FM and Contact Resonance modes assess the viscoelastic response of the sample, that is, the loss modulus or the loss tangent. They also look at its storage modulus or elastic response. This is important to know in a variety of situations which require impact resistance or toughness, which are heavily affected by the material’s viscous properties.
- The Cypher AFM family uses small cantilevers with the AM-FM Viscoelastic Mapping Mode to perform quantitative mapping of the nanomechanical properties of the sample much quicker than is possible using any other method.
- The NanoRackTM Stretch Stage is brought into use in some situations requiring the measurement of materials which are under tensile strain, with the MFP-3D AFM series.
|
Measuring Thermal Properties
Many polymers show very significant changes in properties as the temperature increases. It is important to understand glass transition temperatures and similar characteristics in order to gain a deeper insight into molecular motion. This would enable the evaluation of properties which are critical to their performance such as toughness and impact resistance, and to understand how they are affected by processing or by high temperatures in their operating surroundings.
When AFM measurements are taken at varying temperatures, they provide data on many processes like crystallization, melting, glass transitions and sub-glass transitions. The use of sample heating stages ensures that temperatures can be varied with strict control over the range to be studied for polymer transitions. Figure 8 illustrates how modulus in polyethylene terephthalate varies with temperature, this being a thermoplastic material. Figure 9 reveals the changes in morphology of a microparticle with shape memory as it undergoes heating.
In addition to assessing the changes in form and properties in relation to the temperature, there are also ways to directly analyze heat properties at nanoscale. One is scanning thermal microscopy (SThM), which uses a custom cantilever specially designed to make contact with the sample while monitoring the changes in temperature caused by a local heat source. As it measures the local surface temperature of the sample, image contrast is produced in direct relation to the local conductivity to thermal energy. Figure 10 shows an example of SThM used to image a polymer blend.
Another thermal imaging method is local thermal analysis (LTA), which uses a probe that is heated locally rather than a local sample surface temperature sensor, to heat a nanoscale volume of the sample. With heating or cooling of the sample, there is expansion or contraction in keeping with its local coefficient of thermal expansion. The cantilever deflection reflects this change, generating deflection-temperature curves.
These curves are extremely sensitive when it comes to detecting the beginning of a thermal transition in the sample, and can thus differentiate various materials by detecting their melting or glass transition temperatures. The use of this technique in testing a ternary polymer blend is shown in Figure 11.
.jpg)
Figure 8: Temperature dependence of PET viscoelastic properties – (left) Force curve for polyethylene terephthalate (PET). A 3 second hold segment of constant force was applied between the force loading and unloading cycles. (center) Creep curves of indentation versus time during the force curve hold interval. The curves have been normalized to vary between zero and one, highlighting the increase in relaxation time with temperature. (right) Temperature dependence of instantaneous modulus E1 (pink squares) and long term modulus E2 (black diamonds) obtained by fitting the creep curve to a three-element Maxwell-Voigt model (inset, center). Both E1 and E2 exhibit a dramatic drop in the temperature range between the glass-to-rubber transition temperature Tg ≈ 77 °C and the PET crystallization temperature Tc ≈ 13 5°C due to the film’s semicrystalline nature in this range. Acquired with the MFP-3D AFM and PolyHeater sample stage. Adapted from Ref. 8.
.jpg)
Figure 9: Annealing of shape memory polymer – Tapping mode topography images of a polystyrene (PS) microparticle during annealing. Scan size 12 μm, Z (height) scale 1.4 μm. The PS particle was flattened with a high-temperature, high-pressure nanoimprint lithography (NIL) process and then thinly coated with gold. Imaging began with the particle heated to 80°C. The heater temperature was rapidly increased to 102°C and then increased stepwise, so that it was 106°C after 78 min. and 110°C at 170 min. During annealing the particle diameter decreases and the height increases, recovering the original, pre-NIL spherical shape. A surface wrinkling morphology develops that gives information on recovery dynamics and strain energy release. Applications for micro- and nanoscale polymer particles include drug delivery and electronic packaging; incorporating shape memory effects could lead to many more. Imaged with the MFP-3D AFM. Adapted from Ref. 9.
.jpg)
Figure 10: SThM on PP-PS-PE ternary blend – The sample contained 60% polypropylene (PP), 20% polystyrene (PS), and 20% polyethylene (PE) by weight. In this SThM image, the three components are clearly distinguished, with darker colors corresponding to higher thermal conductivity. As expected from literature values for their thermal conductivity, the oblong regions of PS are brightest (yellow, 0.03 W / m•K), the circular regions of PE are darkest (purple, 0.4 W / m•K), and the surrounding PP matrix is intermediate (orange, 0.12 W / m•K).Imaged with the MFP-3D AFM; scan size 15 μm. Sample courtesy of Dalia Yablon and Andy Tsou, Corporate Strategic Research, ExxonMobil Research and Engineering.
.jpg)
Figure 11: LTA of PP-PE-PS ternary blend – (top) AM-FM Viscoelastic Mapping Mode image of the same sample used in Figure 10. The brighter colors indicate higher modulus. Scan size 6 μm. The black dots indicate points where LTA measurements were made. (bottom) The LTA measurements are color coded with the points in the image and clearly distinguish the different transition temperatures for PS (blue), PE (green) and PP (red). Sample courtesy of Dalia Yablon and Andy Tsou, Corporate Strategic Research, ExxonMobil Research and Engineering.
Asylum AFMs for Thermal Measurements
- All MFP-3D AFMs except for OriginTM are compatible for the PolyHeater (from ambient temperatures to 300°C), PolyHeater+ (from ambient temperatures to 400°C), and CoolerHeater (from -30°C to +120°C) sample stages. These operate in harmony with the Environmental Controller to achieve a closed-loop control for the temperature which is fully programmable.
- Cypher ES AFMs have a Heater (temperatures from ambient to 250°C) and CoolerHeater (0°C to 120°C) sample stages. These accurately regulate temperature without the need for external controllers or other electronic components, or for cooling pumps.
- All MFP-3D family AFMs use state-of-the-art microfabricated silicon probes with the SThM probe holder to achieve SThM temperature and thermal conductivity measurements with higher resolution than Wollaston-wire probes.
- The Zither™ Modulated Thermal Analysis Option available with Cypher and MFP-3D family AFMs (except Origin) allows LTA to achieve a better than 20 nm lateral resolution. The use of proprietary calibration and measurement protocols make the results extremely sensitive but prevent the occurrence of artifacts like those produced by thermal drift.
|
Monitoring Dynamic Processes due to Solvent and Thermal Effects
In an environment that is not at room temperature, the change in morphology and physical properties over time must be understood. When a polymer interacts with a liquid or vapor solvent, the durability and performance of the polymer over the long term can go down. In other applications, the rise in temperature can affect the lifetime or dependability of the device.
Once the solvent concentration or thermal gradients are controlled, the more advanced AFMs such as the Cypher have fast scan rates to monitor dynamic behavior directly, such as degradation or decomposition, chain and brush ordering, formation of lamellae, crystallization and melting. It is possible to obtain movies showing the mechanical or topographical properties if the frame rate is below one minute. In Figure 12, one such instance is seen where the changes in morphology occur when a melted polymer recrystallizes.
It is often difficult to operate in tapping mode using solvents or other liquids due to the multiple resonance peaks occurring as a result of mechanical coupling between the tapping piezo and the fluid. This has been countered using different techniques using various actuation platforms. That is, actuation by photothermal, electromagnetic or other forces that brings the multiple peaks down to a few, making it easier to use tapping mode with more stable results.
.jpg)
Figure 12: Recrystallization of PP – A blend of polystyrene (PS) and polypropylene (PP) was heated to 140°C to melt the syndiotactic PP and then cooled at a constant rate while imaging at one frame per minute. As the sample cools, the continuous PP phase first nucleates and then forms partly ordered, semicrystalline regions. Some of the regions form on top of the PS spherical domains. Imaged with the Cypher ES AFM; scan size 4 μm.
Analyzing Electrical and Functional Behavior
Polymers have unique characteristics and can be manufactured at low costs, rendering them very appealing for a host of applications like flexible electronics, photovoltaics using organic sources, organic LEDs, MEMS actuators and sensors, and data storage devices. At nanoscale, the electrical and mechanical properties of these materials must be well understood, and this becomes more vital as miniaturization of devices proceeds. One particularly important area is finding the correlation between any local heterogeneities and the bulk performance.
There are specialized modes of AFM operation to examine the electrical and electromechanical behavior of nanoscale of polymers which have conductive, semiconducting and dielectric properties. Some of these modes are conductive AFM (CAFM), electric force microscopy (EFM), and Kelvin probe force microscopy (KPFM). These yield electrical data on conductivity, surface potential, photocurrents and work function.
When piezoelectric and ferroelectric polymers are being tested, piezoresponse force microscopy (PFM) is used to analyze electromechanical behavior at nanoscale, such as polarization switching and domain growth. Figure 13 shows how PFM is useful in bringing out fine detail on ferroelectric polymer nanomesas.
.jpg)
Figure 13: Time-dependent domain switching of PVDF-TrFE – (a) Topography and (b) PFM phase images of as-grown polyvinylidene fluoride-trifluoroethylene (PVDF-TrFE) nanomesa. Scan size 600 nm, Z scale 30 nm (height) and 15° (PFM phase; minimum blue, maximum yellow). (c) PFM phase image of the same nanomesa after global switching due to a DC bias of +6 V. (d-f) Time-dependent polarization orientation switching from an applied voltage pulse of -5 V after (d) 1 s, (e) 4 s, and (f) 8 s. The approximate tip position is indicated by the black dot in (a). The high electromechanical activity of PVDF-TrFe makes it attractive in many transducer and sensor applications. Imaged with the MFP-3D AFM. Adapted from Ref. 10.
Asylum AFMs for Examining Functional Behavior
- The ORCATM is a module in Cypher S and MFP-3D AFMs which allows current measurement at sensitive scales for CAFM. It can also make use of an optional amplifier which has low-noise and dual-gain properties, to allow measurements to be taken at high sensitivity over a range of under 1 pA to 10 µA.
- NanoTDDBTM is another unique Asylum mode for time-dependent dielectric breakdown assessment at smaller scales than can be achieved using conventional probes. Here, the tip-sample voltage is kept constant or increased in steps to 220 V until the detection of a breakdown event.
- Asylum has the only commercially available high-voltage PFM mode that can handle up to 220 V for use by the MFP-3D AFM and the Cypher family AFMs. All AFMs from Asylum have integrated software that offers very sensitive PFM measurements, with the Dual ACTM Resonance Tracking (DARTTM) which has been patented by Asylum, or the unique Band Excitation option.
|
Increase Output with Asylum AFMs
This article provides a number of examples to show how powerful the use of Asylum AFMs can be in analyzing polymers, whether for scientific or engineering purposes. Newer developments have made AFMs able to acquire images to visualize a number of different physical properties and behavior as well as morphology. Asylum AFMs incorporate many remarkable functionalities, such as high spatial resolution, rapid scanning, several operating modes, and so on, which will enable many more characterizations of polymers.
References
- P. Samori, M. Surin, V. Palermo, R. Lazzaroni, and P. Leclere, Phys. Chem. Chem. Phys. 8, 3927 (2006).
- L. Sawyer, D. Grubb, and G. Meyer, Polymer Microscopy, 3rd Edition (Springer, New York, 2008).
- M.E. McConney, S. Singamaneni, and V.V. Tsukruk, Polym. Rev. 50, 235 (2010).
- H. Schönherr and G.J. Vancso, Scanning Force Microscopy of Polymers (Springer Laboratory, Heidelberg, 2010).
- N.T. Lawrence, J.M. Kehoe, D.B. Hoffman, C. Marks, J.M. Yarbrough, G.M. Atkinson, R.A. Register, M.J. Fasolka, and M.L. Trawick, Macromol. Rapid Comm.31, 1003 (2010).
- T. Gkourmpis, C. Svanberg, S.K. Kaliappan, W. Schaffer, M. Obadal, G. Kandioller, and D. Tranchida, Eur. Polym. J. 49, 1975 (2013).
- S. Kienle, T. Pirzer, S. Krysiak, M Geisler, and T. Hugel, Faraday Discuss.160, 329 (2013).
- C.A. Grant, A. Alfouzan, T. Gough, P.C. Twigg and P.D. Coates, Micron 44, 174 (2013).
- L.M. Cox, J.P. Killgore, Z. Li, Z. Zhang, D.C. Hurley, J. Xiao, and Y. Ding, Adv. Mater. 26, 899 (2013).
- P. Sharma, T.J. Reece, S. Ducharme, and A. Gruverman, Nano Lett.11, 1970 (2011).
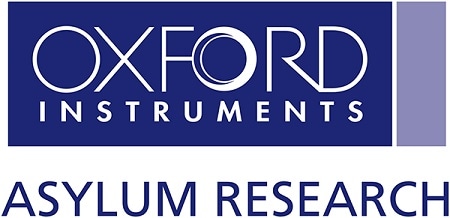
This information has been sourced, reviewed and adapted from materials provided by Asylum Research - An Oxford Instruments Company.
For more information on this source, please visit Asylum Research - An Oxford Instruments Company.