Introduction
The force of interaction between colloidal particles plays an important role on the stability and rheology of the ceramic slurries and forming of the green bodies. The well-known DLVO theory, which states that the net energy of interaction is a sum of van der Waals and electrical forces, provides a theoretical tool for predicting these forces quantitatively [1, 2]. The van der Waals force depends mainly on the bulk properties of the interacting bodies, not so much on the solution chemistry. The electrostatic force, however, is affected markedly by the solution characteristics and is manipulated to control rheology and stability. It arises from the electrical double layer which develops due to the different chemical activities of the ions in solution and on the solid’s surface. In oxide-water systems, the proton concentration is the main parameter which determines the surface charge, hence the electrostatic force, through such reactions as Al—OH0 + H+ → Al—OH2+ or Al—OH0 → Al—O- + H+.
The DLVO theory has been shown to represent the interactive behavior of colloidal systems well. One of the best proofs is the Shulze-Hardy rule which can be directly derived from the DLVO theory [3]. However, its testing with direct measurements of the interparticle forces has been possible after the introduction of the Surface Force Apparatus (SFA) [4-6]. SFA can only be used with transparent substrates such as mica, glass or quartz since it relies on interference fringes. Also, this procedure practically has no lateral resolution [7].
The Atomic Force Microscopy-Colloid Probe Method (AFM-CPM), which does not suffer from such shortcomings, has been finding wide use in recent years for interparticle force measurements [8, 9]. In this method, the bending of a tiny cantilever which carries an attached colloid particle at its free end is measured optically by a laser-photo detector system as it is brought closer to a substrate. The amount of deflection can be directly related to the interactive force between the colloid and the substrate if the spring constant of the cantilever is known. Using Derjaguin's approximation, the force of interaction can be translated to the energy of interaction if the colloid’s diameter is much larger than the gap between the surfaces. The forces in the order of few picoNewtons can be detected by AFM which theoretically has a quantum level resolution (better than 10-24 J) [10]. The reader is directed to the pioneering work by Ducker et al. [10, 11] and reviews by Parker [11], Claesson et al. [12], Senden [13] and Hodges [14] for details.
The direct force measurements in recent years have shown that the oxide-water interface displays an unusual behavior which cannot be accounted for by the DLVO theory, especially at distances shorter than 10 nm [15-21]. Several other works suggest the presence of a long-range attractive interaction (>100 nm) in the case of hydrophobic surfaces [22-24]. For the oxide-water system, one explanation was the presence of a repulsive ‘hydration force’ at separations below 5 nm. Karaman et al. [18] using AFM-CPM observed that plasma-oxidized alumina-sapphire surfaces always demonstrated repulsive forces within pH range 3-6.5. Though their force-distance curves agreed well with the DLVO theory at separations larger than 3-5 nm, no distinctive energy barrier and a primary minimum were visible. The fact that the surfaces repelled at intermediate pH where the sapphire and α-alumina surfaces should be oppositely charged [27] is also surprising. This behavior was attributed to the presence of a thick repulsive hydrated layer (15 nm) on the surface at basic pH due to the swelling of the alumina. However, no force curves were provided for the basic pH range. They also suggested a ‘thin’ gel at lower pH values which conflicts with the always-repulsive interaction energy observed at pH 3.5.
In this study, systematic normal and lateral force microscopy measurements were carried out between α-alumina colloid probes and 0001 sapphire surface using AFM-CPM in strongly acidic, neutral and strongly basic solutions. The purpose was to ascertain more definitely the effect of pH on interactive forces in a model oxide-water system, to obtain a correlation between these forces and the hydration behavior and to determine how the measurements correlate with the DLVO theory.
Materials and Method
A 0001-sapphire substrate (from As One Corporation, Japan) and á-alumina powders (from Admatechs Co., Japan) were used for the force measurements and for the FTIR and TG-DTGA studies. The isoelectric points of the á-alumina powders and the sapphire were 8.9 and 5. 1 [25]. The sapphire was atomically smooth with a Ra value of 0.11 nm with a maximum peak-to-valley distance of 0.92 nm. Micro-contact angle measurements on UV-treated sapphire surface
gave no contact suggesting that the surface was perfectly clean and hydrophilic after treatment.
The rectangular tipless cantilevers (TL-FM-50 from Nanosensors, Switzerland) were used to prepare the colloid probes. The cantilevers were individually tested for their dimensions, resonant frequencies and Q factors in air at room temperature to determine the normal spring constant for each cantilever [26-28]. Though there are methods to determine the lateral spring constant of a rectangular cantilever28, presence of a glue layer fixing the colloid on the cantilever and of the particle itself introduce unknowns which would make such constant unreliable. Therefore, the lateral force data in this paper is reported in terms of deflection, which is equivalent to the force normalized for the spring constant since deflection=lateral force/lateral spring constant. Such normalization requires that the same colloid probe is used throughout a given set of lateral force measurements for meaningful comparison of data.
A repeated decantation-ultrasonification process at pH 3.5 was employed to isolate individual α-alumina colloid probes and to clean their surfaces. A micromanipulator with 0.1 μm resolution (Model M501-1202-M from Suruga Seiki Co. Ltd., Japan) was employed to pick, position and fix these colloids on the cantilevers using a rapid type epoxy glue (Araldite AR-R30-Nichiban Co. Ltd., Japan). Some representative pictures are given in Figure 1.
A Scanning Probe Microscope (SPA 400, Seiko, Japan) with a liquid cell was used for the normal and lateral force measurements. The liquid cell, the sapphire and the colloid probes were all treated with UV-plasma for 10 minutes (PL16-110D from Sen Light Corp., Japan), washed with ethanol, distilled water and finally with experimental solutions copiously. The whole assembly was immersed in the experimental solution 10 minutes prior to each measurement. Five normal force measurements were obtained at the center and the corners of a 1 μm by 1 μm square area on the sapphire surface. This procedure was repeated 4 times on the corners of a larger, 20 μm by 20 μm area, resulting in 20 force measurements for each test. The lateral force measurement was obtained immediately after each normal force measurement over the same locations. However, a given lateral force measurement was repeated 10 times on a single location at different distances from the surface to differentiate the effect of probe-surface separation on the frictional force. The approach speed of the probe was 30 nm/sec in the normal mode; the drag length and speed were 100 nm and 50 nm/sec in the lateral mode.
Force measurements yield raw data in the form of piezo translation-cantilever deflection signals. These data need to be converted to actual force-distance curves. Figure 2 shows the raw data and the resulting force-distance curve and the algorithm used in the conversion process. In the case of lateral force measurements, the deflection signal was directly used with special attention to using the same colloid probe for the whole set of measurements.
The full numerical solutions of the Poisson-Boltzmann equation which satisfy the surface potentials on both surfaces at each separating gap result in potential profiles as a function of distance from each surface. The electrostatic pressure between the surfaces, which is the interaction force per unit area, can then be directly calculated from these potential profiles for each separating gap. The surface potentials employed in these calculations were +90 mV for both surfaces at pH 3.5, +40/-40 mV at pH 7.7 and -90 mV for both surfaces at pH 12 based on the zeta potential measurements of the α- alumina colloids and the literature data for the 0001-sapphire surface [25].
á-alumina powders treated in solutions of pH 3.5 and 12 (in 10-4 M KCl) were analyzed by FTIR (Spectrum GX FTIR-DRIFT from Perkin Elmer, USA) and TG-DTGA (2000S from Mac Sciences Co. Ltd., Japan) to assess the water at the α-alumina surface.
Results and Discussion
The separation vs. normal force curves determined by AFM-Colloid Probe Method are given in Figure 3 for pH values of 3.5, 7.7 and 12.0. The figure also includes the interaction energy calculated from the force using the Derjaguin’s approach. The results display major differences in the interaction energy for the three pH values studied. At pH 3.5, where both surfaces are positively charged, a very well defined repulsive barrier exists at a separation of about 10 nm. At closer approach, the force becomes quickly attractive. For pH 7.7, the interaction is attractive at all separations for the two oppositely charged surfaces. At pH 12, the interactive force does not show neither a repulsive barrier nor an attractive region and is always repulsive for all separations. Similar behavior was also reported in the literature [15-19] and was attributed to a repulsive force caused by a layer of hydrated oxide at the surface. Preliminary results of the lateral forces measurements at pH 3.5 and 12 show that the frictional forces differ markedly at acidic and basic pH values (Figure 2) such that the frictional force is much smaller in the case of pH 12 where a hydration layer on the surface is conjectured.
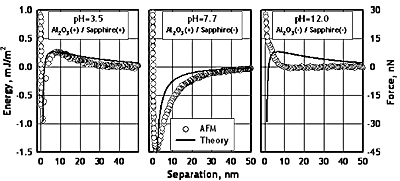
Figure 1. Normal interaction forces between the á-alumina colloid probe and the sapphire substrate at different pH (C0=10-4 M KCl; T=25 oC; kn=2.8 N/m; R=5.4 μm).
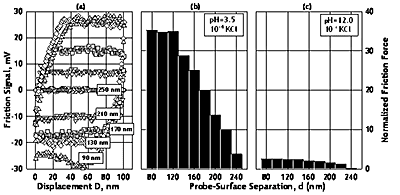
Figure 2. The lateral interaction forces between the á-alumina colloid probe and the sapphire substrate as a function of probe-surface separation at different pH (C0=10-4 M KCl; T=25 oC; R=5.4 μm).
Presence of such a hydrated layer was indicated by FTIR-DRIFT spectra of the α-alumina powders treated in pH 3.5 and pH 12 solutions for 24 hours (Figure 3). The OH-stretching region between 2800-3800 cm-1 was deeper for pH 12, suggesting that the α-alumina surface contained more H-bonded water at basic pH. A broad hydroxyl stretching region for several alumina powders was also observed using FTIR-DRIFT in the literature [29]. TG and DTGA analyses of the same samples showed that almost all the water in the sample was lost before 100oC for pH 3.5, whereas the pH 12 sample retained some water at temperatures above 100°C, supporting the existence of some amount of more strongly bonded water (Figure 4). Chemisorbed water on α-alumina was also reported in the literature [30, 31].
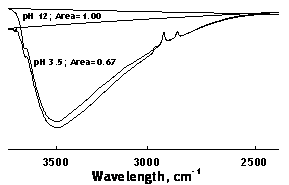
Figure 3. FTIR-DRIFT spectra (normalized with respect to the pH 12 spectra) of the H-bonded water region for the α-alumina samples treated in pH 3.5 and pH 12 solutions for 24 hours.
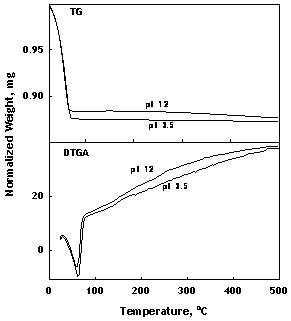
Figure 4. TG and DTGA data for the α-alumina samples treated with pH 3.5 and pH 12 solutions for 24 hours.
Conclusions
Normal and lateral AFM-Colloid Probe force measurements of α-alumina colloids and 0001-sapphire substrate were carried out in solutions of pH 3.5, 7.7 and 12. For normal force, the interaction of protonated, positively charged α-alumina surfaces showed a well-defined repulsive barrier at 10 nm followed by an attractive minimum. The force was always attractive at pH 7.7 for the oppositely charged surfaces as expected. In basic pH, where the both surfaces were negatively charged, the interactive force was short-ranged and always repulsive such that an attractive minimum did not exist. For lateral force, the friction seemed considerably smaller at basic pH values, most probably due to the presence of the short-range repulsive forces observed. It seems that presence of a hydration layer is possible on the alumina surface at basic pH values. Such a layer could hinder the closer approach of the surfaces and result in the observed behavior in both normal and lateral interactions. These findings have important implications in the stability and rheological behavior of ceramic slurries as well as the forming of green bodies.
References
1. L. E. Shoemaker, “Joining Techniques for Ferritic Oxide Dispersion Strengthened Alloys”, proceedings of the International Conference on Trends in Welding Research, Gatlinburg, TN, USA, ASM International, 371-377, May 1986.
2. E. Lugscheider, H. Schmoor and U. Eritt, “Optimization of Repair-brazing Processes for Gas Turbine Blades”, Brazing, High Temperature Brazing and Diffusion Welding, Deutscher Verlag fur Schweisstechnik GmbH, Dusseldorf (Germany), 259-261, 1995.
3. W. F. Gale and E. R. Wallach, “Microstructural Development in Transient Liquid Phase Bonding”, Metall. Trans A, 22A, 2451-2457, 1991.
4. M. C. Chaturvedi, O. A. Ojo and N. L. Richards, “Diffusion Brazing of Cast Inconel 738 Superalloy”, AZo Journal of Materials online, DOI: 10.2240/azojomo0123, 2005. (This paper was also published in print form in Advances in Technology of Materials and Materials Processing, 6 [2] (2004) 206-213).
5. H. Nakagawa, C. H. Lee and T. H. North, “Modelling of Base Metal Dissolution Behavior during Transient Liquid-Phase Brazing”, Metall. Trans. A, 22A, 543-555, 1991.
6. L. J. Park, H. J. Ryu, S. H. Hong and Y. G. Kim, “Microstructure and Mechanical Behavior of Mechanically Alloyed ODS Ni-Base Superalloy for Aerospace Gas Turbine Application”, Advanced Performance Materials, 5(4), 279-290, 1998.
7. ASM Handbook, “Alloy Phase Diagrams”, Metals Park, Ohio, vol. 3, p 313, 1992.
8. T. I. Khan and E. R. Wallach, “Transient Liquid Phase Diffusion Bonding and Associated Recrystallization Phenomenon when Joining ODS Ferritic Alloys”, J. Mater. Sci., vol.31, 2973-2943, 1996.
Contact Details |