For the purposes of performing pulse and temperature-programmed chemisorption methods, which are used to characterize and test catalysts in-situ, the PID EFFI (Efficiency) microactivity reactor can be reconfigured and connected to a mass spectrometer.
Called the 'Micro Catalyst Characterization and Testing Center' (MCTC), this is an innovative research tool which enables numerous catalyst characterization experiments, as well as activity testing at pressures which range from atmospheric pressure to as high as 200 bar (depending on the configuration of the instrument).
It is possible to use characterization techniques such as temperature-programmed desorption, oxidation, and reduction as a reference point before the catalyst’s performance is tested. Important information regarding the behavior of the catalyst’s active life and deactivation can then be gathered, thanks to the ability to follow the catalyst’s transient activity online.
It is possible to obtain crucial information relating to the parameters which may cause a loss in the catalyst’s activity by re-characterizing the catalyst's post-activity. Accurate and important information regarding catalytic behavior for certain applications can be obtained through the combination of performance testing and characterization in just one apparatus.
The Instrument
Displayed in Figure 1 is the MCCTC configuration of the PID EFFI. This is designed specifically for catalytic testing and catalyst characterization, with a view to determining the specimen’s activity and selectivity.
The instrument’s key components include the following: three six-port valves which dictate flow pathways and pulse through the reactor; mass flow controllers (MFCs) for the active and carrier gases; a quadrupole mass spectrometer which enables online analyses of gas mixtures; and a heated reactor zone which is capable of reaching temperatures as high as 1,100 degrees centigrade using a variety of ramping rates.
The liquid evaporator (for cases which involve liquid feeds), together with all the valves and plumbing, are encased in a hot box which is heated to 200 °C. This ensures the quality mixing of gases and prevents condensation within the system.
The patented Liquid/Gas separator is used to cool heated vapors which condense at atmospheric conditions, and to then separate them from the gas phase. It is possible to collect these liquid products for further studies. The dry gas undergoes composition analysis in the mass spectrometer.
.jpg)
Figure 1. Flow Diagram of the MCCTC Connected to a Mass Spectrometer.
Experiment 1: Temperature-Programmed Reduction (TPR)
In addition to determining the effect of the catalyst support on the dispersion of active metal(s), a temperature-programmed reduction is used to characterize the reducible species which exist in the catalyst.
In this instance, the H2 pressure’s effect on the catalyst’s reduction profile was analyzed by obtaining TPR profiles at two very different pressures; at 1 bar and 25 bar. A 50:50 ratio of copper (II) oxide and silver (I) oxide was used in this study, with 80 mg of each being utilized.
For species reduction, a 50 sccm flow of a 10% hydrogen-balanced nitrogen mixture was used. As a carrier gas and a diluting agent, a flow of 100 sccm nitrogen was supplied. In order to generate complete TPR profiles, the furnace temperature was ramped at a rate of 10 °C per minute up to 500 °C.
In a similar manner to how the majority of TPR studies are carried out, the initial step involved a temperature-programmed reduction being performed on the silver and copper oxide mixture at atmospheric pressure.
Figure 2 displays the atmospheric pressure TPR profile. Figure 2 shows a sharp inverted peak at 163 °C and a broader inverted peak at 320 °C. These represent the activation temperatures of the silver oxide and copper oxide respectively.
The second step involved performing the same TPR analysis at a pressure of 25 bar. Figure 3 displays the 25 bar pressure TPR profile. This includes a sharp inverted peak at 150 °C and a broader inverted peak around 250 °C. The inverted peaks represent the decrease of H2 downstream of the catalyst which is being reduced.
Ag2O + H2 ----> 2AG + H2O
CuO + H2 ----> Cu + H2O
Hydrogen is consumed during the reduction. Following the chemical reactions, it combines with the oxygen atoms in the metal oxide. As expected, it is evident that the high H2 pressure over the catalyst noticeably decreases the reduction temperature. A higher driving force for the interaction of oxides and hydrogen is provided by this increased pressure, particularly in relation to the porous catalyst supports.
As reducing catalysts at lower temperatures significantly decreases the chance of sintering, ultimately enabling higher catalytic activity, this principle is extremely valuable in the industry. TPR is a bulk reaction, which means that it is possible to make a quantitative analysis with the mass spectrometer data in order to establish the total amount of reducible species present in a catalyst.
Experiment 2: Pulse Chemisorption (PC)
An analytical technique that is often used to establish the dispersion (or accessible active species present on the surface) of a catalyst is pulse chemisorption (PC). This works by pulsing an active gas like CO or H2 (as in this case) onto the catalyst at room temperature and determining the quantity of active gas adsorbed.
In this instance, a six-way valve was used to pulse the active gas. This valve has a 0.5 ml STP loop in intervals of roughly three minutes. In order to guarantee complete filling within a minute, the 10% hydrogen-balanced nitrogen mixture was introduced into the loop at a flow rate of 20 sccm.
.jpg)
Acting as a carrier gas, nitrogen at atmospheric pressure was fed through at a constant rate of 50 sccm. The studied catalyst constituted around 800 mg of 0.5 wt% Pt/Al2O3.
The data which was acquired from the mass spectrometer over the course of the experiment is displayed in Figure 4. Five separate pulses are represented by the five different peaks. The final three peaks are identical. These represent pulses occurring once the catalyst has been saturated. Their purpose is to provide a baseline, enabling a back-calculation of the quantity of gas which is adsorbed in the initial two pulses.
Nearly all of the active gas gets adsorbed in the initial pulse, while some are adsorbed during the second pulse. The total quantity of adsorbed gas can be figured out by integrating the peaks and combining this data with a known pulsed volume. The following equation is used to determine the present dispersion of the catalyst.
Where D% is the percent dispersion, Fs is the stoichiometry factor (in this case 2 for diatomic hydrogen), Wa is the atomic weight of the active metal, Vads is the total volume of gas chemisorbed, 195 AMU for platinum, Vmol is the molar volume of the adsorptive (22.414 liters per mol), and M% is the percentage of active metal by weight (as grams of metal per gram of sample).
A well-known standard that is used for Micromeritics Chemisorption instruments such as the Autochem II 2920, the studied catalyst (0.5 wt% Pt/Al2O3) has a reported dispersion of 35% ± 5%. 36% was yielded by the percentage dispersion which was calculated using data collected by the mass spectrometer and MCCTC.
More active species are adsorbed when the PC experiment is performed at increased pressures. Consequently, there will be a proportionally higher percentage of dispersion of the catalyst. It is commonly understood that catalysts acting at atmospheric pressure demonstrate a higher activity than that which they indicate at the increased pressures which are required for industrial chemical reactions.
Further to the above, the PC technique can determine additional parameters, like the size of the active particles and the active metal surface area. Sintering or growth of the active particle is indicated by large active particle size. In general, the catalyst’s activity decreases in line with an increase in active particle size.
.jpg)
Figure 2. TPR Profile performed at atmospheric pressure.
.jpg)
Figure 3. TPR Profile performed at 25 bar pressure VadsFs Wa VmolM% D%= •100•100.
Additional Catalyst Characterization Experiments Using the MCCTC
Temperature-Programmed Desorption (TPD)
In this technique, the quantity of gas which is desorbed from a catalyst’s surface during a linear temperature ramp, once an active gas has saturated the surface, is measured. This method evaluates the strength of the interactions of adsorbed molecules which are bonded to the active species. It also quantifies surface coverage.
Further to the above, this method can determine the proportion of adsorbed molecules that are present on the surface of the catalyst up to the reaction temperature. For instance, the catalyst would be able to perform the hydrogenation reaction if hydrogen is retained on a catalyst like platinum up to the desired reaction temperature.
Figure 5 illustrates this analysis, as it displays a TPD profile of hydrogen on platinum collected on the Autochem II 2920. A thermal conductivity detector (TCD) is used by the Autochem II 2920 in order to quantify the amount of hydrogen present in the exhaust stream. The MCCTC, combined with a mass spectrometer, provides the same TPD profile.
Occurring here at three separate temperature peaks, the increasing signal intensity indicates the release of hydrogen from the catalyst’s surface. The catalyst’s different active sites are indicated by the multiple peaks shown. Peaks that occur at higher temperatures reflect sites with higher adsorption enthalpies.
It is possible to perform TPD at increased pressures with the use of the MCCTC. However, this should only be done if the catalyst was reduced at the same pressure.
.jpg)
Figure 4. Pulse chemisorption profile of H2 on 0.5 wt% Pt/Al2 O3.
.jpg)
Figure 5. TPD profile of H2 on a platinum supported alumina catalysts.
Temperature-Programmed Oxidation (TPO)
The main purpose of this technique is the determination of the degree of reduction of the active species present on the catalyst. The number of regeneration cycles a catalyst can undergo prior to completely deactivating can be estimated by combining this technique with TPR. De-NOx catalysts like TiO2 and V2O5 can also be characterized by TPO.
In the industry, these catalysts are widely used to transform dangerous NOx contamination into other components that are non-pollutant. Generally, nitrogen and water can be produced safely, as these catalysts promote the reaction of NH3 with NOx.
A typical TPO profile of the pre-reduced de-NOx catalyst V2O5 is shown in Figure 6. Three oxidation peaks are included in the TPO profile, two of which are well defined at 500 °C and 860 °C respectively, while the third is a 'shoulder' near 960 °C.
The stability of the reduced species which will be active for the reaction is indicated by peaks appearing at such elevated temperatures. The catalyst in question had a superb yield for a de-NOx catalyst, where activity testing reported a 95% conversion rate.
.jpg)
Figure 6. TPO Profile of V2O5, a de-NOx Catalyst.
Conclusion
Combining nearly all of the simple catalyst characterization techniques with catalytic activity testing, the MCCTC is an innovative piece of equipment. Overall costs are reduced by this combination, as the requirement for numerous pieces of equipment is eliminated by the versatility of a single instrument.
It is extraordinarily valuable that this instrument can characterize different catalysts at different experimental temperatures and pressures, as the catalyst can undergo preliminary characterization and testing with the use of the same industrial reaction conditions.
Prior to activity testing, the catalyst’s behavior at the pre-set experimental conditions can be predicted by the fact above. This enables the determination of the cause of catalyst deactivation through post-activity testing characterization. Money and time are ultimately saved by the user if they elect to test and characterize activity with the use of only one system..
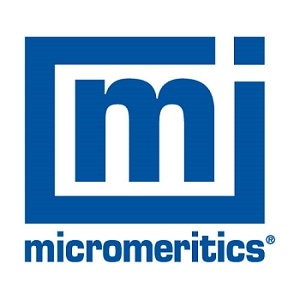
This information has been sourced, reviewed and adapted from materials provided by Micromeritics Instrument Corporation.
For more information on this source, please visit Micromeritics Instrument Corporation.