Developments in semiconductor technology have driven growth across an extensive range of areas in everyday life, with continuous advances being made delivering state-of-the-art phones, computers, automobiles, and innovations in information technology development and artificial intelligence. All of this has significantly contributed to the improved quality of life for the global population.
O-PTIR spectroscopy offers exceptional capabilities for many advanced failure analysis applications, including:
- 30x improvement on submicron IR spatial resolution when compared with conventional FTIR/QCL microscopy
- Delivers unique co-located fluorescence microscopy for rapid identification of organic contaminants and faster time to data
- High-quality spectra (particle shape/size and surface roughness independent) with no dispersive/scatter artefacts that can be searched directly in commercial/custom FTIR transmission or ATR libraries
- Non-contact, non-destructive, reflection (far-field) mode measurements with minimal sample preparation
- O-PTIR offers superior measurement of high-resolution, dark-colored, light-sensitive samples, which usually face limitations when existing Raman and FTIR techniques are used.
- Simultaneous O-PTIR and Raman measurement of devices for complementary and confirmatory results with improved speeds relative to time to data and improved data confidence
These developments continue to fuel growing investment into the technology and manufacture of semiconductor devices at both industrial and academic research levels. Improvements in technology, including new materials, increasingly narrow semiconductor linewidths, manufacturing processes and device structures, are all propelling the requirements for new metrology and inspection methods for in-line, offline, and failure analysis.
The Failure Analysis (FA) process and technologies allow manufacturers to establish what causes failure in semiconductor devices. The analysis includes a diverse range of processes and measurement equipment to discover the underlying cause and prevent any future reoccurrences.
FA measurement techniques may vary relating to analysis time, data acquisition, chemical specificity, spatial resolution, sample preparation requirements, complexity, automation capabilities, and cost. For instance, scanning electron microcopy, when paired with energy dispersive X-Ray (SEM-EDX) spectroscopy, can offer exceptional spatial resolution, but with only elemental chemical information, as displayed in figure 1.
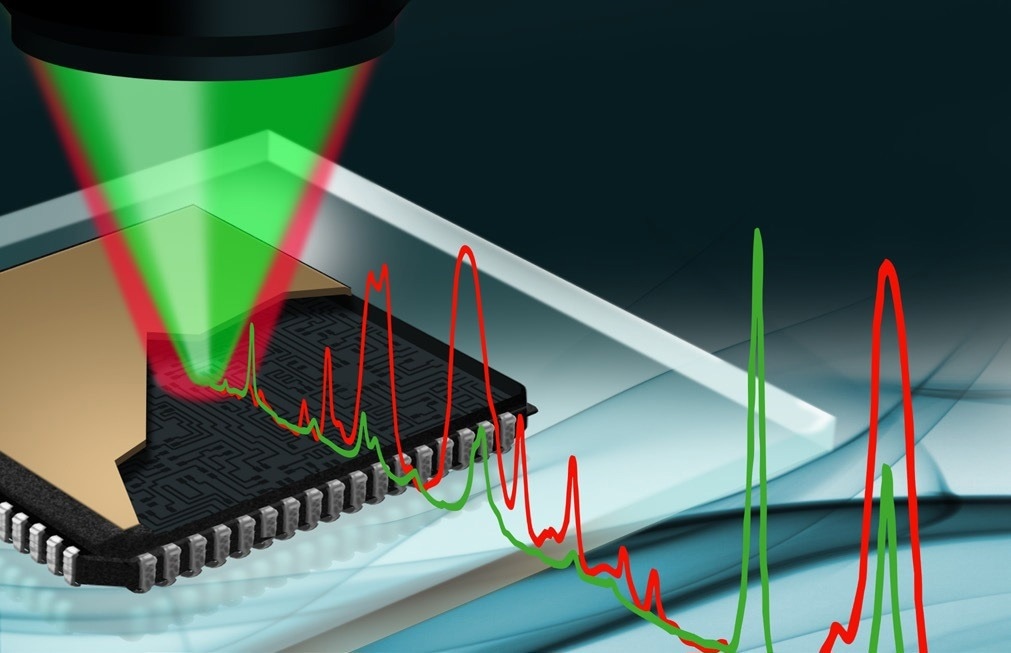
O-PTIR Provides unique measurement capabilities for semiconductor failure analysis. Image Credit: Photothermal Spectroscopy Corp
Generally, elemental information alone remains insufficient when instilling high confidence, as unknown identification and organic molecular information is also required to determine unknowns with a greater degree of certainty.
Technologies such as Infrared (IR) and Raman microspectroscopy are well-established techniques in FA that offer organic molecular information.
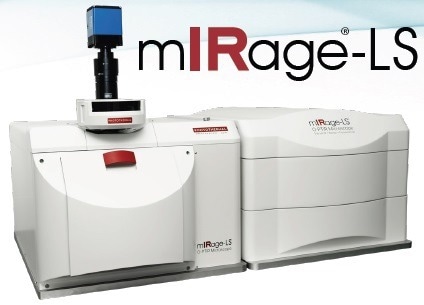
mIRage-LS sub-micron IR spectroscopy and imaging system. Image Credit: Photothermal Spectroscopy Corp
Relating to chemical imaging, the capacity of Raman and FT-IR spectroscopy equipment is considered wide reaching with spatially resolved chemical characterization capabilities that have been applied to a diverse range of application areas: device degradation, foreign materials analysis, formulation errors raw material impurity qualification; all of which may lead to early failure of components and devices within semiconductor and electronic products.
However, traditional FT-IR microscopy is limited in its spatial resolution and sensitivity for FA. At the same time, the sensitivity of Raman microscopy is often too low for numerous samples due to autofluorescence issues. It can cause laser-induced sample damage, which can be more notable in darker materials.
An innovative technique, Optical Photothermal Infrared (O-PTIR) microscopy, can circumvent the limitations above, while preserving the complementary nature of Raman and IR.
O-PTIR also has stable integration with both Raman spectroscopy and fluorescence microscopy to identify specific organic contaminants and guide the subsequent IR measurement. Figure 1 exhibits a graphical representation of various conventionally applied analytical FA techniques, plotted against spatial resolution and chemical information content.
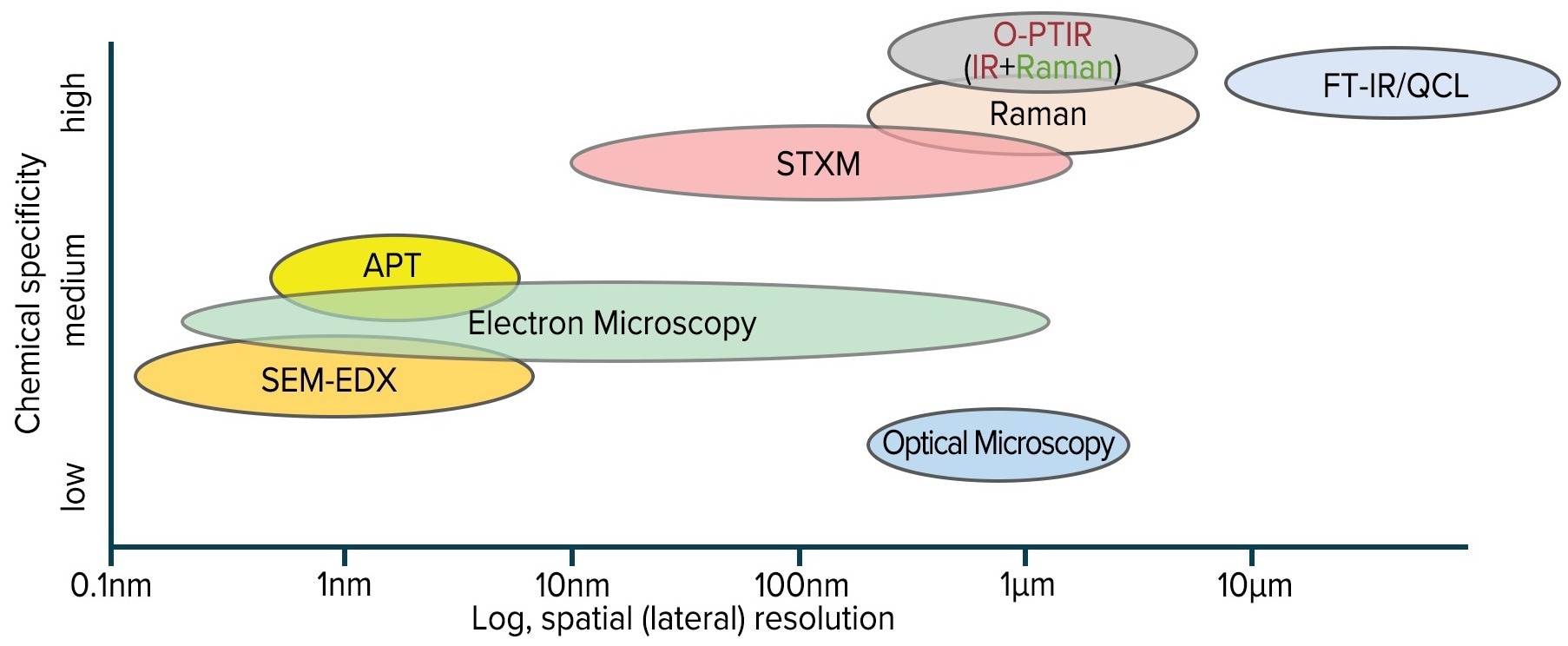
Figure 1. Spatial resolution and relative chemical specificity of correlative methods. Image Credit: Photothermal Spectroscopy Corp
This article describes the innovative O-PTIR approach and how it is integrated with Raman and fluorescence techniques for failure analysis applications on the mIRage-LS platform.
Optical Photothermal IR (O-PTIR) Spectroscopy with Simultaneous Raman Spectroscopy
By illuminating the sample with a mid-IR pulsed tunable quantum cascade laser (QCL) and measuring infrared absorption indirectly with a visible laser beam (532 nm), the O-PTIR technique can overcome the IR diffraction limit affiliated with conventional IR microscopy techniques.
When tuning the QCL laser to a wavelength that excites molecular vibrations in the sample, absorption can be observed, producing photothermal effects, for example, sample surface expansion and a variation in refractive index.
The visible probe laser measures the photothermal response, focused to sub-micron spot size, via a modulation induced in the scattered light, as displayed in figure 2. To acquire an IR spectrum, the IR laser can sweep the entire range in less than two seconds.
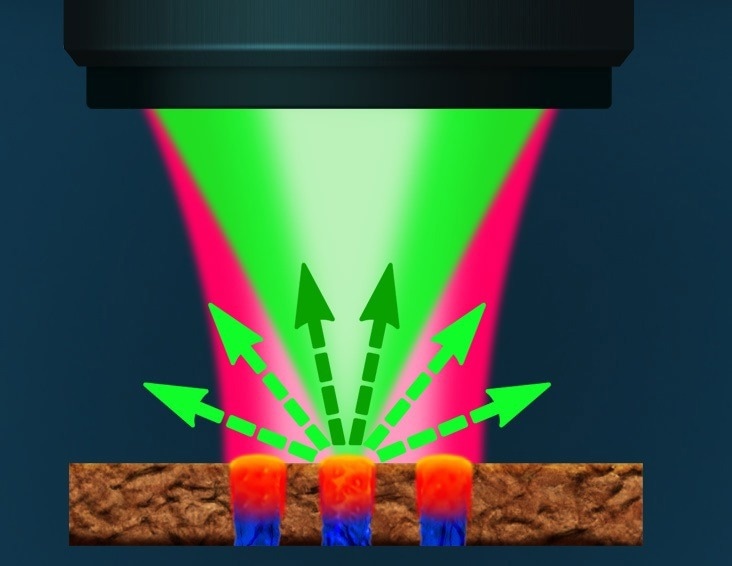
Figure 2. A pulsed tunable, IR source is focused on sample. Absorbed IR light causes the sample to heat up, creating a photothermal response. The visible laser probe within the diameter of the IR light measures the photothermal response from the sample upon IR absorption. Image Credit: Photothermal Spectroscopy Corp
Moreover, the visible probe laser also produces scattered Raman light from the same measurement area. When gathered together, it enables simultaneous acquisition of both IR and Raman spectra, with the same submicron spatial resolution, at the exact location.
A schematic diagram demonstrating this optical beam path is displayed in figure 3 below. With simultaneous measurement capabilities, researchers can leverage the complementary nature of both techniques and deliver confirmatory spectral information for highly accurate measurements.
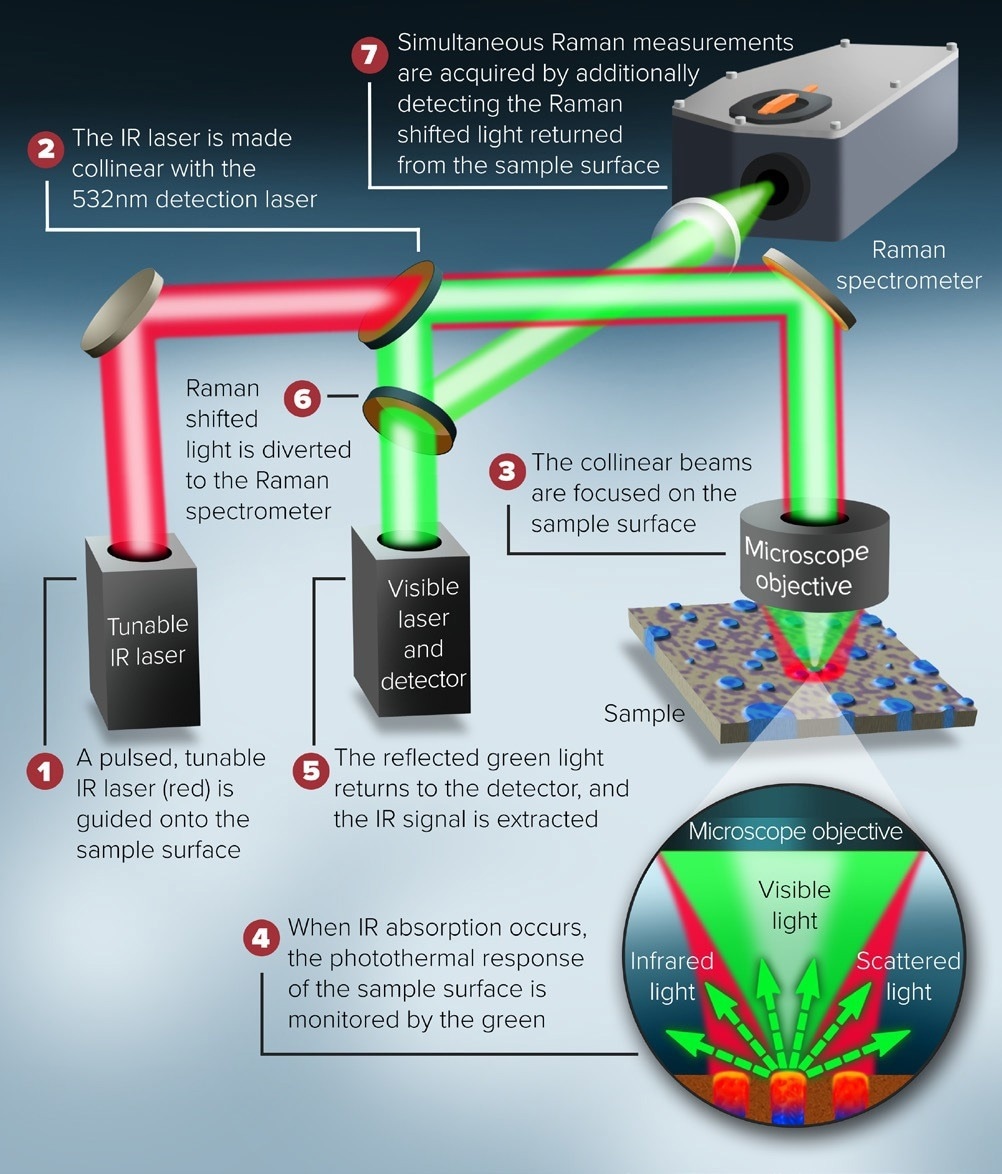
Figure 3. Schematic representation of the beam paths for the generation of simultaneous IR and Raman. Image Credit: Photothermal Spectroscopy Corp
Figure 4 displays the variable spatial resolutions found in traditional IR microscope systems over the conventional infrared range using two IR objectives of 0.5 or 0.7 NA versus the constant spatial resolution of the O-PTIR technique with the application of 0.78 NA objective (Kansiz, 2020).
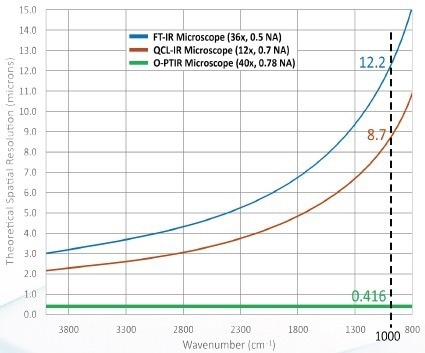
Figure 4. Spatial Resolution comparison between conventional FTIR, QCL-equipped IR microscope and the O-PTIR pump-probe infrared microscope. Image Credit: Photothermal Spectroscopy Corp
The O-PTIR technique provides wavenumber independent spatial resolution across the whole mid-IR range due to using a wavelength probe beam fixed at 532 nm.
mIRage-LS Principal of Operation: O-PTIR and Fluorescence Microscopy
The layout of the system for a combined O-PTIR sub-micrometer IR microscope, co-located with fluorescence microscopy, is shown in figure 5. The pulsed IR beam is directed through the underside of the sample when set to counter the propagating mode. The fluorescence and visible probe beam paths are co-located using the microscope objective.
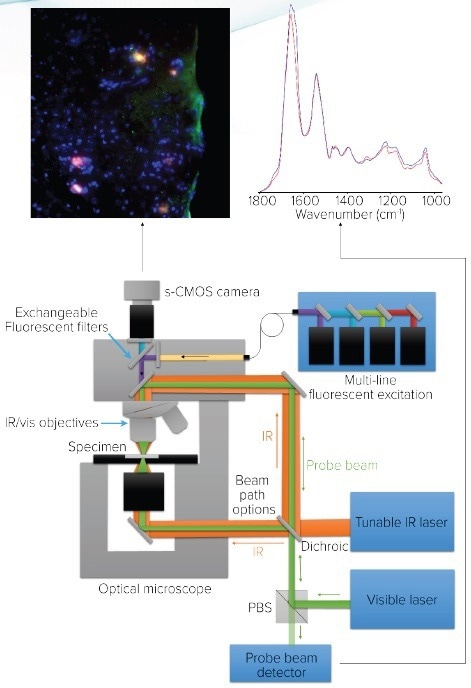
Figure 5. Microscope schematic for O-PTIR and co-located fluorescence microscopy. Image Credit: Photothermal Spectroscopy Corp
Simultaneous IR+Raman Spectral Searching with 2D Search Result Representation with KnowItAll®
One of the main objectives of any FA process is determining the chemical identification of the unknown material. As a result, the final step after spectral acquisition is to search against a spectral database.
Usually, when searching for IR spectra, Raman spectral would be searched against an IR spectral library and, separately, against a Raman spectral library. The user would then carefully evaluate the two so-called “hitlists” for IR and Raman spectra.
With the advances made towards simultaneous submicron IR+Raman spectral acquisition, as displayed in figure 6, not only are the IR and Raman spectra acquired simultaneously, but now the spectral search of both IR and Raman spectral also occur at the same time, with just a single click from the data acquisition software.
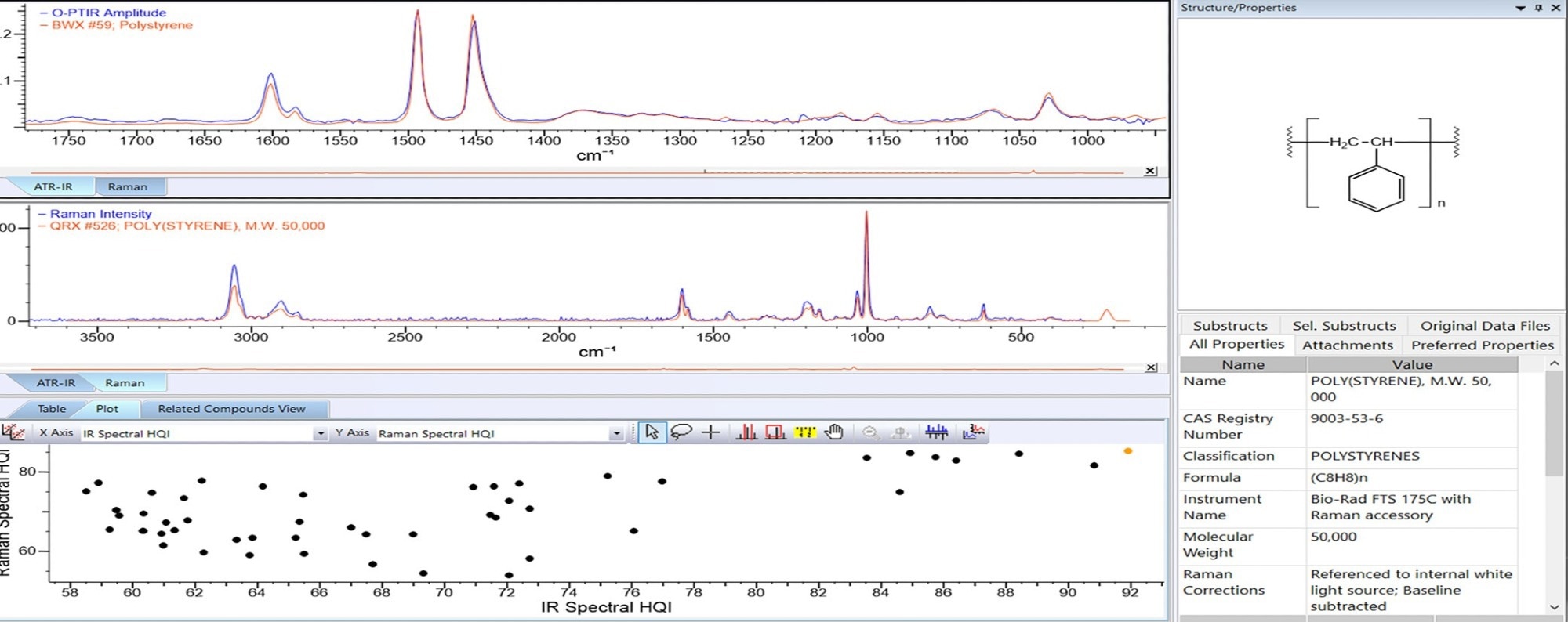
Figure 6. Example of a simultaneous IR+Raman search result from a simultaneous IR+Raman measurement. Screen capture from the Wiley’s KnowItAll® spectral search engine shows the IR spectrum (top spectral pane) with the unknown spectrum in black and reference library spectrum in red. The Raman spectra are plotted in the middle pane with again, the unknown spectrum in black with the reference library Raman spectrum in red. The lower pane consists of the 2D scatter plot showing the best result as the datapoint in the upper right corner (orange point) with both the highest IR HQI and highest Raman HQI. Image Credit: Photothermal Spectroscopy Corp
Moreover, to assist in assessing the results, the IR and Raman hit lists are displayed in a 2D scatter plot, with the IR Hit Quality Index (HQI) plotted on one axis and the Raman HQI plotted on the other axis. Figure 6 shows an example of a simultaneous IR+Raman search result for a standard plastic.
When evaluating a 2D scatterplot, the best result can be found in the upper right corner of the plot, where the result suggests both a high IR and high Raman HQI, providing a confirmatory and complementary aspect. The benefits of this novel approach are two-fold:
- Productivity and ease-of-use gains via a simple one-button spectral export and loading into KnowItAll®, readily displaying the data to the analyst for searching and providing answers.
- The increased accuracy and confidence users can achieve in the data that comes with IR+Raman spectral, which offers both complementary and confirmatory data, i.e. the IR search result confirms the Raman and vice-versa.
High-Value Failure Analysis Applications with O-PTIR and Combined Techniques
High resolution chemical imaging of failed device features
Exhibited in Figure 7, O-PTIR overcomes the current associated challenges of infrared spectroscopy techniques with an example outlining the dark suspected creep in the underfill (UF) between two metal cross-sectioned surfaces (Zulkifli, 2022).
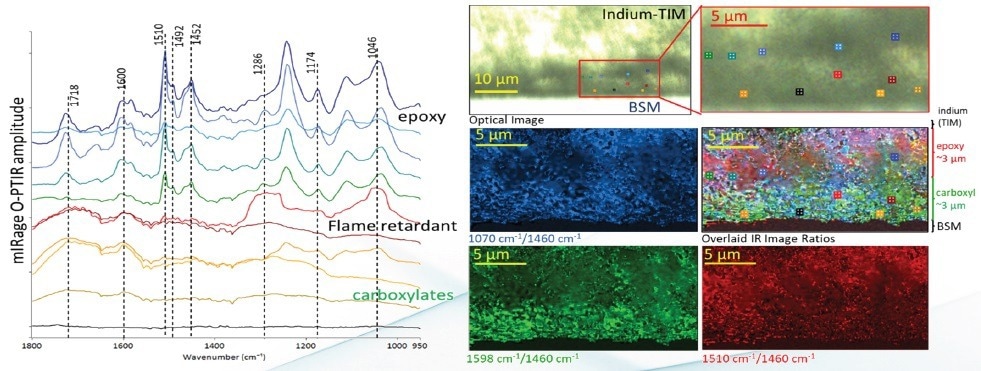
Figure 7. Submicrometer O-PTIR spectroscopy and imaging analysis of a suspected creep specimen. Image Credit: Photothermal Spectroscopy Corp
With the O-PTIR technique, several components can be separated and identified spectroscopically. Here, it is determined that the top 3 µm of the dark contamination is an epoxy component, which is typically the organic binder component in underfill materials. The bottom 3 µm layer seems to possess considerable amounts of carbon and carboxylates; the latter may have derived from oxidized cellulosic matter [Madorsky, 1958].
Such details of an unprecedented nature offer investigative insights into locating the source of the contamination, raw materials, or errors in the process. Conversely, traditional FT-IR microspectroscopy could not provide practical information from the same specimen (Zulkifli, 2022).
A significant advantage found when using O-PTIR for this measurement is that the sample preparation uses standard chemo-mechanical polishing techniques, suggesting a significant time saving over more intricate techniques, such as time-consuming, laborious focused ion beam sectioning.
Failure analysis of a capacitor device with simultaneous O-PTIR and Raman
Polished cross-sections of capacitors with dark organic metal multilayer structures are typical examples of a sample type demonstrating the main benefits of simultaneous O-PTIR and Raman analysis.
Displayed in Figure 8, a typical cross-section of an electrolytic capacitor (E-cap): a thin, 10 µm layer of dielectric material centrally located between the metal casing and center electrode. The organic material absorbs infrared broadly and strongly, in line with a dark-colored conductive polymer. Therefore, minimal infrared light returns to the infrared detector in an FT-IR measurement.
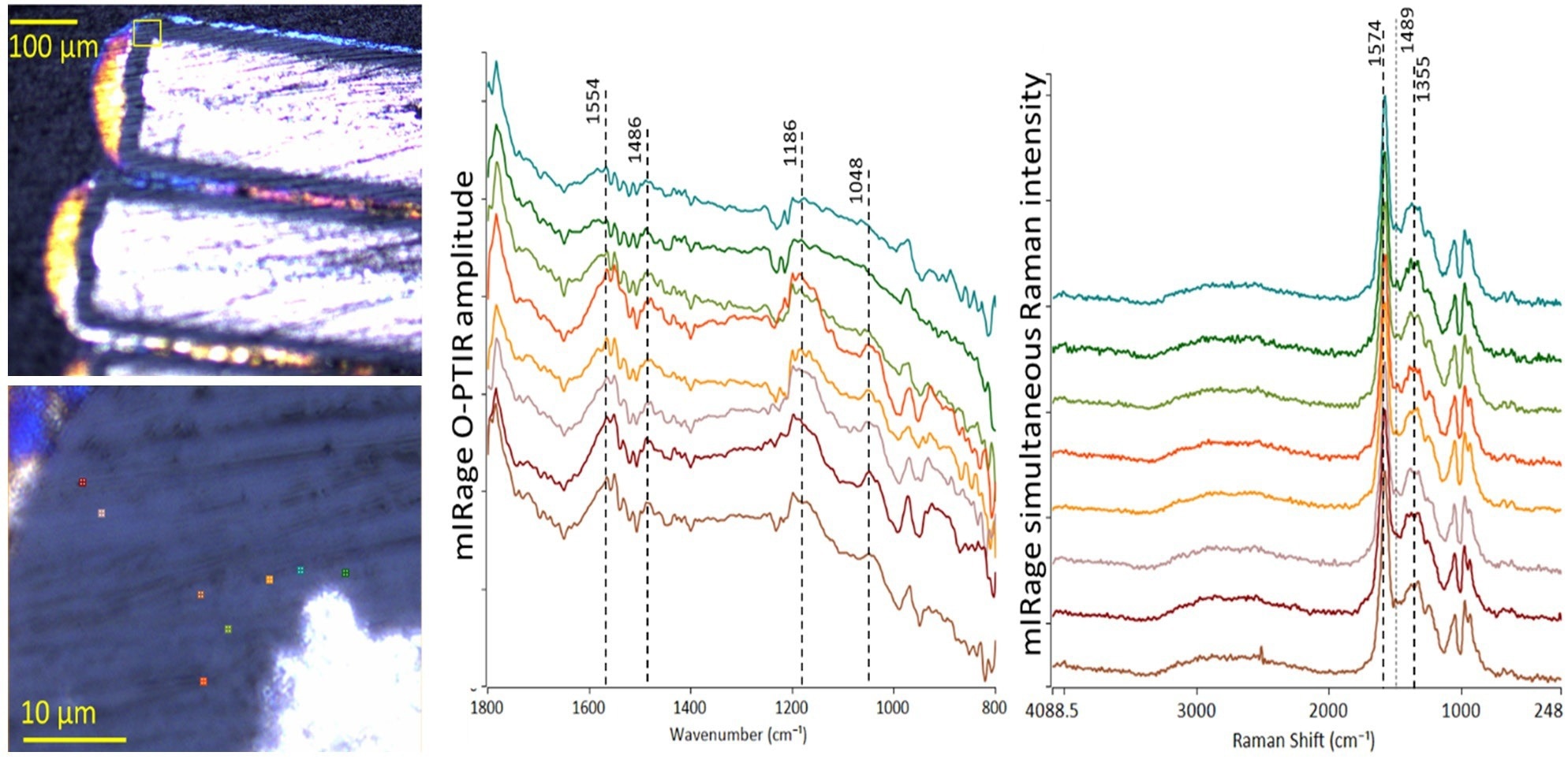
Figure 8. Cross-section analysis of an organic dielectric in a normal capacitor. Image Credit: Photothermal Spectroscopy Corp
Such an experiment usually produces poor, noisy FT-IR spectra results with few intelligible spectroscopic details (Zulkifli, 2022). Measuring the IR spectrum leveraging an ATR accessory can be problematic due to the levels of complexity when it comes to achieving sufficient optical contact between the internal reflection element (IRE) and the hard metallic material, which can also damage IRE.
The O-PTIR technique can counter these challenges by acquiring spectra from a sub micrometer sample spot size in a non-contact reflection measurement. The transmission-like IR spectral band shapes can be attributed to the smaller sampling area probed by the visible laser and localized sensing of photothermal effect.
Figure 8 displays the strong O-PTIR baseline, confirming the broad, strong IR absorption properties of the dark dielectric layer. O-PTIR spectra exhibits spatially resolved spectroscopic differences, which have extended IR absorption profiles provoked by the presence of graphitic carbon.
The dielectric’s chemistry is most consistent using a polyphenylene derivative (Furukawa, 2002). The sub micrometer O-PTIR and simultaneous Raman acquisition facilitates definitive simultaneous characterization of both polar and non-polar species at the same spot at the same time. Here, the strong Raman shifts at 1574 cm-1 and 1355 cm-1 align with graphite (Tunistra, 1970), verifying observations made in the corresponding O-PTIR spectra.
Conversely, the chemical signature of a cracked E-cap cross-section is considerably different from that of the typical specimen. While it can be seen that a solid spectral baseline offset is present in Figure 9, O-PTIR spectral band shapes differ significantly, and a new 1100 cm-1 signal emerges at several marker locations.
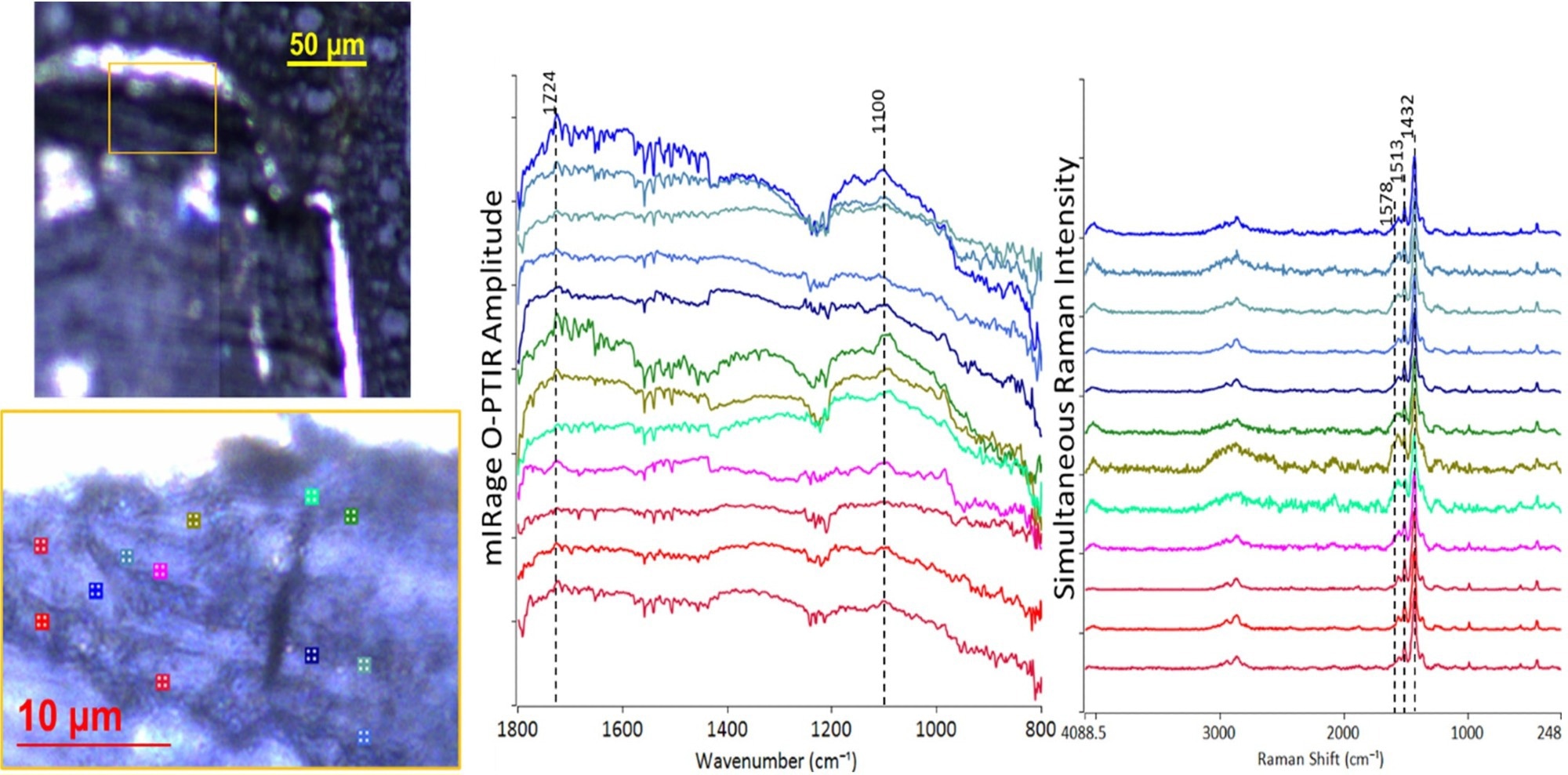
Figure 9. Cross-section analysis of organic dielectric in a failed capacitor (Zulkifli, 2022). Image Credit: Photothermal Spectroscopy Corp
The 1100 cm-1 band is potentially due to a polyethylene glycol derivative being present; this is a known reagent for enhancing the performance of electrolytic capacitors (Xiong, 2012). The instrument’s simultaneous Raman feature generates a sharp spectral signature consistent with PEDOT:PSS (Chang, 2014), likely the primary component.
If conducted separately, identifying unknown compositions would have been challenging, if not impossible, but simultaneous analysis can be a highly effective tool using the combined sub micrometer O-PTIR and Raman. The rough topography of the cross-section does not hinder the identification process.
These results from the combined approach demonstrate that the organic dielectric layer from the two suppliers have varying chemical compositions and that the composition of the cracked E-cap may have been one cause of the failure.
O-PTIR for measurement of dark-colored light sensitive materials
Determining chemical compositions of foreign matter in an open circuit makes up one of the most common, longstanding industrial problems in microelectronics failure analysis. Unfortunately, the layers in these devices are typically narrower than the diffraction limits of micrometer-sized infrared wavelengths, pushing the resolving power of conventional FT-IR microscopes to their limits.
Raman microscopy could be a suitable alternative spectroscopic technique to measure these samples, but the dark coloration of the sample usually results in excessive heating and fluorescence, which, in turn, can cause sample damage.
Reducing the laser power to lower the heating effect results in Raman spectra with SNRs that fall below the levels needed to record usable chemical information from the tested area and impractically long measurement times.
Sample burning or melting becomes a more significant risk since a stronger sample illumination is required to achieve high signal-to-noise measurements. Raman scattering occurs once per one-million incident photons, with high signal-to-noise measurements requiring strong sample illumination.
Figure 10 shows samples that can easily melt at typical Raman laser power levels, destroying a sample (Anderson, 2022). A standard dark sample – fig.10(a) measured with Raman can lead to sample damage, as seen in figure 10(b) as well as poor quality Raman spectra exhibited in figure 10(c).
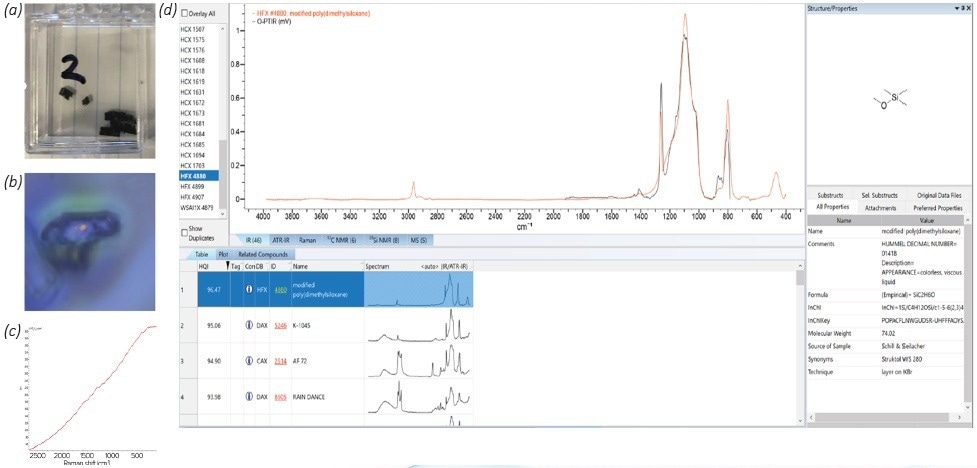
Figure 10. (a) A dark specimen routinely occurring in microelectronics FA applications. (b) Damage caused to dark sample by Raman power in the milliwatt ranges needed for adequate signal-to-noise. (c) Poor Raman spectral quality as sample browns under milliwatt power. (d) High quality IR data collected and searched against commercially available databases (e.g. KnowItAll®) with >96% HQI using APD with visible power used in the microwatt ranges. Image Credit: Photothermal Spectroscopy Corp
Even when no sample damage occurs at low milliwatt laser power levels, Raman spectra would be useless due to strong auto-fluorescence baselines that can occur when illuminating strongly colored materials.
Using an avalanche photodiode (APD) detector to collect O-PTIR data enhances and addresses inherently low sample reflectivity from dark colored and rough samples, while ensuring high spatial resolution. As shown in Figure 11, the APD exhibits high sensitivity at low probe laser illumination levels far below the threshold of the sample’s laser damage.
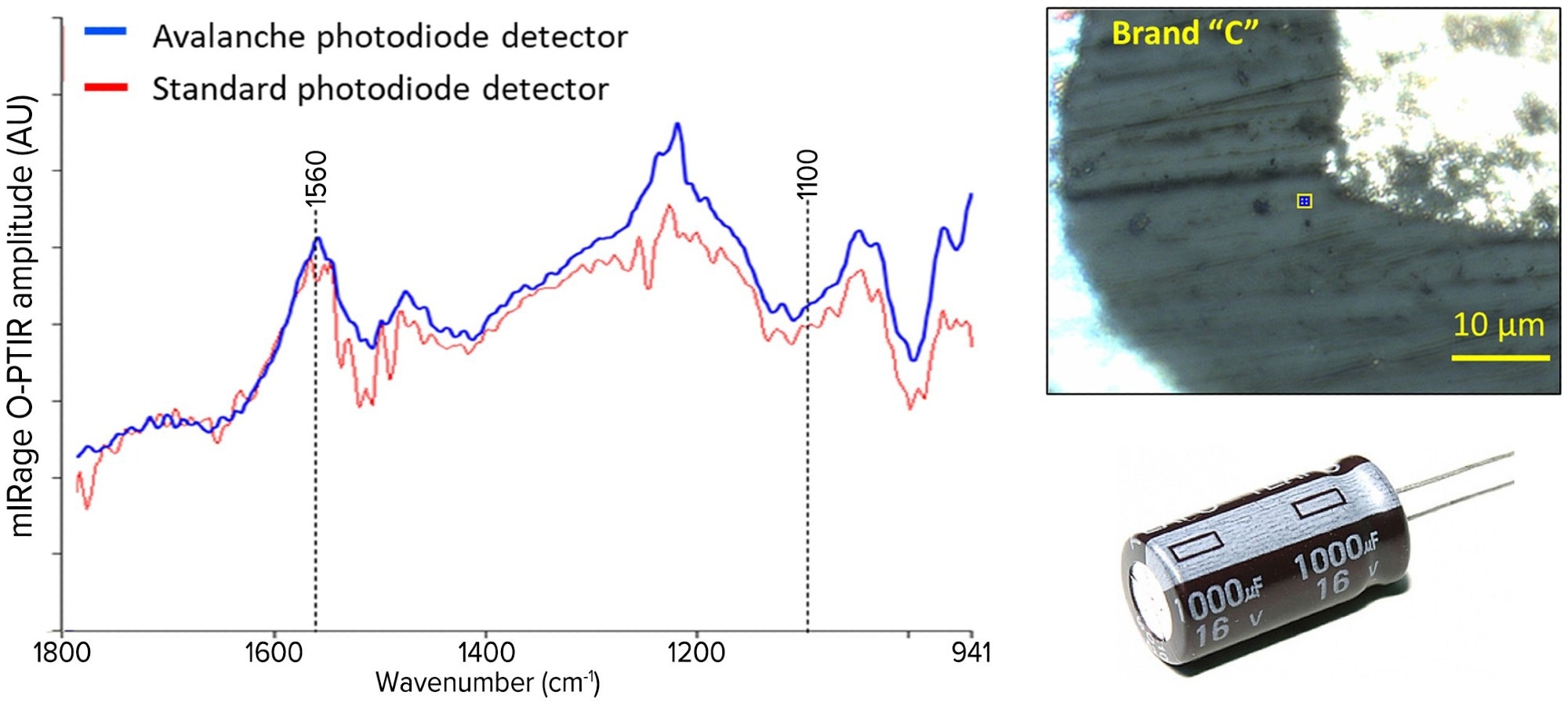
Figure 11. Optical image and O-PTIR spectra obtained at the blue marker location from the cross-section of Brand C electrolytic capacitor; blue spectrum taken with an APD and red with a standard photodiode detector. Image Credit: Photothermal Spectroscopy Corp
Compared to the standard detector, using APD yields improved SNR data, offering significantly improved clarity and confidence to material differentiation. Moreover, the rough topography of such samples does not hinder the acquisition of high SNR infrared absorption spectrum when using mIRage O-PTIR with an APD detector.
Fast identification of regions of interest using co-located fluorescence and O-PTIR
The O-PTIR technique has proven successful in establishing the chemical identity of complex samples, which had not been previously possible with the limited spatial resolution and sensitivity conventional infrared microspectroscopy offers. The noncontact operational nature of Raman microscopy is usually limited by strong autofluorescence (AF) and poor spectral sensitivity inherently present in several materials.
Rapid and efficient data acquisition is extremely important in a fast-paced testing facility with high throughput. The brightfield or optical camera in the mIRage instrument generates a high-quality visible image from which the user can readily highlight regions at high speeds for subsequent OPTIR measurements. However, sometimes the contrast in the visible image may be insufficient when alerting or guiding the user to where the known or unknown defects are located.
As previously demonstrated, the spatial composition can be complex; it would be beneficial and time-saving to have localization and differentiation of species within the region of interest (ROI) before collecting the first O-PTIR (and simultaneous Raman) spectrum or image.
Fluorescence (FL) images can be acquired by exciting the region of interest (ROI) with varying visible wavelengths and identifying the radiation re-emitted at longer wavelengths. Fluorescence imaging has been in use for a long time in material science applications.
A particular molecule's excitation and emission wavelengths are directly related to its inherent electronic states but would not impact O-PTIR data acquisition (Qin, 2020; Chen, 2022). Resultingly, widefield FL images can first map out the ROI before the acquisition of spatially resolved chemical information by submicron O-PTIR.
Figure 12 demonstrates an innovative application of fluorescence imaging for determining the dark ROI surrounding a solder joint, which meant it could not bond with an aluminum pad.
The brightfield (BF) image displayed in figure 12(a) shows how users are able to locate the metallic surfaces from the underfill, but it was not possible to determine any chemical variation within the nearby organic matter. When the same ROI is illuminated separately using 497 nm, 578 nm, and 628 nm excitation lines, as displayed in figure 12(b), relative FL intensities over the ROI could be quickly recorded.
The FL image overlaid in figure 12(b) shows chemical contrast between the various areas of the ROI, readily providing locations for acquiring O-PTIR spectra. These locations could not be discerned in the BF image alone, as shown in figure 12(a). It can be seen that the chemistry of the underfill differs extraordinarily compared to the contamination near the gap.
The intensities of the changes are noteworthy, indicating location targets for acquisition of O-PTIR spectra. The FL images' utility can correspond to an O-PTIR image at 1732 cm-1, as shown in figure 12(c).
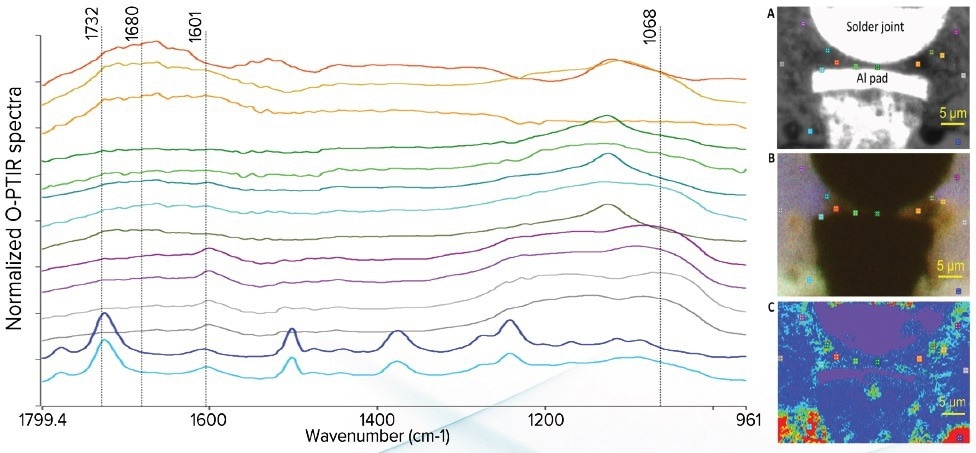
Figure 12. Fluorescence-guided O-PTIR analysis of the cross-section of a non-wetting solder joint due to the presence of organic matter on the aluminum pad; Inset A: brightfield image (A); Inset B: image after overlaying individual fluorescence images from excitation wavelengths of 497 nm (blue), 578 nm (green) and 628 nm (red); Inset C: O-PTIR image taken at 1732 cm-1; all data is taken with the APD detector. Image Credit: Photothermal Spectroscopy Corp
The broad absorption around 1680 cm-1, in combination with the warm-colored markers, are in accordance with carboxylates. The increases in absorption baseline arise from disordered carbon (Lo, 2021).
At the more distant purple zone of figure 12 (b), the gray markers symbolize normal spectra consistent with the silica-enriched epoxy underfill. The purple spectra close to the solder joint are within the area that is slightly dimmer than the bulk underfill on the FL image, which corresponds well with the O-PTIR image for greater IR absorption due to the presence of carbon.
Moreover, the brightest FL region emerges from the polyimide solder resist, which is backed up by high-quality O-PTIR spectra in accordance with polyimide (blue). Again, it is possible to equip the multimodal mIRage-LS O-PTIR instrument with co-located FL to work with dark and colored contamination far below visible laser damage thresholds, usually less than 0.1 mW. An ultra-sensitive avalanche photodiode (APD) detector could easily achieve high spectral signal-to-noise.
Conclusion
O-PTIR on the mIRage and mIRage-LS platforms is an innovative technique that can circumvent many limitations when using traditional FTIR microscopy and spectroscopy techniques, including its submicron IR spatial chemical resolution, allowing the extended use of IR for critical failure analysis-related applications.
O-PTIR enables faster time-to-data than traditional IR and can function in reflection mode with no scattering artifacts requiring zero or virtually zero sample preparation. APD detection with O-PTIR circumvents a significant limitation found in existing Raman spectroscopy techniques with colored and dark samples, which usually burn or generate low SNR data.
O-PTIR acquired with an APD detector with probe beam powers in the microwatt regime enables the collection of spectral data at submicron resolution that would typically be impossible to collect.
O-PTIR can also be paired with Raman spectroscopy to offer simultaneous Raman and IR imaging and spectroscopy at the same measurement location.
The combination delivers complimentary and confirmatory results, while accelerating the time to data significantly. Unknowns ID in FA further improves when paired with simultaneous IR and Raman spectral searching for more precise identification.
Moreover, with the mIRage-LS platform, O-PTIR can be collocated with fluorescence microscopy for enhanced visualization of specific contaminants for additional chemical analysis with O-PTIR.
References and Further Reading
P. R. Griffiths, J. A. de Haseth. (2007) “Fourier Transform Infrared Spectrometry” 2nd Ed. 2007. DOI: 10.1002/047010631X
Kansiz, et. al. (2020) “Optical Photothermal Infrared Microspectroscopy with Simultaneous Raman– A New Non-Contact Failure Analysis Technique for Identification of <10µm Organic Contamination in the Hard Drive and other Electronics Industry,” Microscopy Today, May 2020, 26. DOI: 10.1017/S1551929520000917
S. L. Madorsky, V. E. Hart, S. Straus. (1958) “Thermal Degradation of Celulosic Materials.” J. Research of the National Bureau of Standards. 60, p. 2853.
IPFA 2022 Best Paper Award
S. Zulkifli, B. Zee, M. K. F. Lo. (2022) “Novel Submicron Spatial Resolution Infrared Microspectroscopy for Failure Analysis of Semiconductor Component.” Proceeding of International Symposium on the Physical and Failure Analysis of Integrated Circuits (IPFA) 2022. Conference Paper #20.
F. Tuinstra, J. L. Koenig. (1970) “Raman Spectrum of Graphite.” J. Chem. Phys., 53, p. 1126. DOI: 10.1063/1.1674108
Y. Furukawa. (2002) “Vibrational Spectroscopy of Conducting Polymers” in J. M. Chalmers, P.R. Griffiths. “Handbook of Vibrational Spectroscopy” Wiley and Sons Ltd, Chicester.
S. Xiong, L. Zhang, and X. Lu, (2012) “Conductivities enhancement of poly(3,4-ethylenedioxythiophene)/ poly(styrene sulfonate) transparent electrodes with diol additives,” Polymer bulletin (Berlin, Germany),, 70, p. 237. DOI: 10.1007/s00289-012-0833-8.
S. H. Chang, C.-H. Chiang, F.-S. Kao, C.L. Tien, C.-G. Wu. (2014) “Unraveling the Enhanced Electrical Conductivity of PEDOT:PSS Thin Films for ITO-Free Organic Photovoltaics.” IEEE Photonics Journal., 6, p. 8400307. DOI: 10.1109/JPHOT.2014.2331254
M. Lo, M. Kansiz, E. Dillon, J. Anderson, C. Marcott. (2021) “Simultaneous, Submicron Infrared and Raman Microspectroscopies for Effective Failure and Contamination Analyses.” Proceeding of International Symposium on the Physical and Failure Analysis of Integrated Circuits (IPFA) 2021. Paper #136. DOI: 10.1109/ IPFA53173.2021.9617346
Qin, Z. et. al. (2020) “Spontaneous Formation of 2D/2D Heterostructures on the Edgesof 2D Ruddleden- Popper Hybrid Perovskite Crystals,” Chem. Mater. 32, p. 5009. DOI: 10.1021/acs.chemmater.0c00419.
Chen, C.K. et. al. (2022) “A portable purification system for the rapid removal of microplastics from environmental samples,” Chem. Eng. J. 2022, 428, p. 132614. DOI: 10.1016/j.cej.2021.132614.
J. Anderson et al. (2022) “Overcoming challenging Failure Analysis sample types on a single IR/ Raman platform.” ISTFA 2022: Conference Proceedings from the 48th International Symposium for Testing and Failure Analysis. DOI: 10.31399/asm.cp.istfa2022p0237.
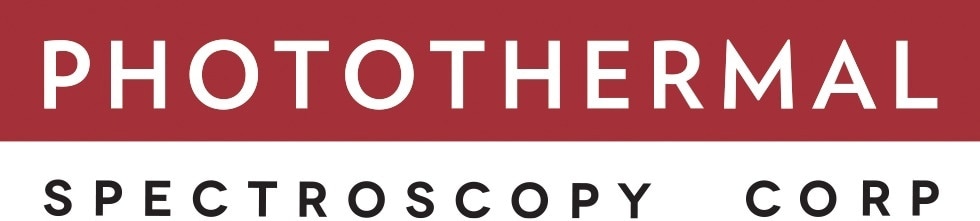
This information has been sourced, reviewed and adapted from materials provided by Photothermal Spectroscopy Corp.
For more information on this source, please visit Photothermal Spectroscopy Corp.