In this interview, AZoM Speaks to Dr. James Sagar, Strategic Product and Applications Manager at Oxford Instruments, about how benchtop NMR can be used to optimize battery electrolyte performance and quality.
Can you give our readers an introduction to the services that Oxford Instruments provide to the battery industry?
At Oxford Instruments we have a strong focus on batteries. we supply a range of analytical solutions to the battery industry, with uses from initial exploration and raw materials processing to the manufacturing of components and their quality control.
We are the leading supplier of electron microscopy-based analysis solutions which are used by our customers to assess the quality of battery raw materials and powders for battery electrodes as well as for high-end research aimed at developing novel materials for solid-state batteries. We allow our customers to understand the details of how electrochemical processes can affect the performance of battery materials using nanoscale surface characterization with our atomic force microscopes.
The focus here, however, is on our Nuclear Magnetic Resonance (NMR) spectrometers which address quality control and product development of the current-carrying liquid electrolytes.
What is the difference between a broadband benchtop NMR system and a traditional NMR setup?
When most people think of NMR, they think of a large cylinder in its own room that requires the use of liquid cryogens and expertly trained operators. With benchtop NMR, we shrink that technology down into an instrument that sits on a bench in a typical lab, albeit at a reduced magnetic field strength. With these compact, lower-cost systems, we can now more efficiently perform many of the same experiments previously requiring a traditional high field NMR system.
Broadband benchtop NMR is an incredibly powerful technique for next-generation batteries. Broadband means that a single instrument can be used to analyze the wide range of elements of interest in battery electrolytes. The technology can be used for new formulation development, in quality control, and to predict electrolyte performance within end product battery systems.
What instrument performance and other considerations impact the choice of benchtop NMR system?
Ideally, a benchtop instrument should operate in any standard lab at room temperature without liquid cryogens. The instrument should not require a dedicated, experienced, or highly trained operator - users should be able to step in and acquire data whenever and wherever they need it. Finally, its dimensions must allow it to fit on a bench, be transported around on a trolley, or placed in an inert glovebox to allow characterization of air-sensitive battery materials.
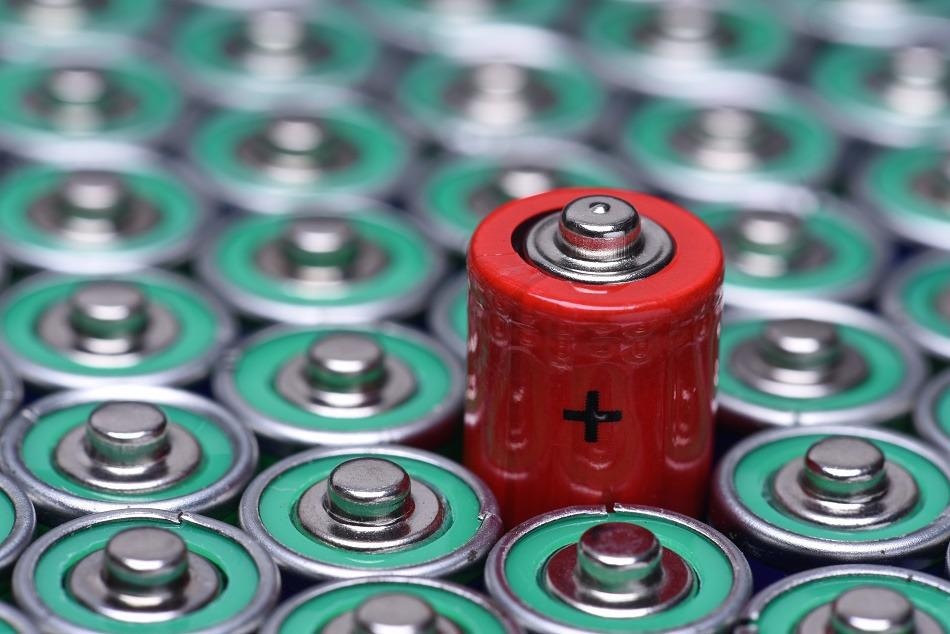
Image Credit: Flegere/Shutterstock.com
In terms of performance, the instrument’s spectral resolution should discriminate between all peaks of interest in your spectra. You need sufficient sensitivity to detect all key species in your materials plus the stability to repeatedly make accurate measurements.
For electrolyte characterization, it is advantageous to be able to analyze all the relevant chemical nuclei in a formulation to ensure the best possible understanding of the materials. This requires a true “broadband” system.
The potential to exploit pulsed-field gradients is important in some advanced experiments. These enable quantification of diffusion coefficients, ionic conductivity, and transference (how the current-carrying ions migrate as a function of their charge). Lastly using variable temperature control to investigate electrolytes over common battery operating ranges can also be important.
How does the X-Pulse benchtop NMR spectrometer help meet these needs?
X-Pulse is our latest benchtop NMR spectrometer. It has better than 0.35 Hz spectral resolution at the half-height of a peak to resolve complex proton spectra at 60MHz field strength.
The X-Pulse is uniquely the only benchtop NMR instrument with broadband multinuclear capability. That allows us to collect spectra from the wide range of nuclei present in electrolytes - everything from lithium to fluorine, phosphorous, boron, sodium, hydrogen, and carbon. We can control the temperature of a sample between 20°C to 60°C, typical operating conditions for most batteries.
The standard pulsed-field gradients can measure the self-diffusion of anion and cation species and therefore understand key physical properties. The physical dimensions of the X-Pulse mean it can be installed in any lab, transported on a trolley, or even potentially integrated into a glovebox. The high stability resulting from the magnet delivers the precise data repeatability required particularly in quality control.
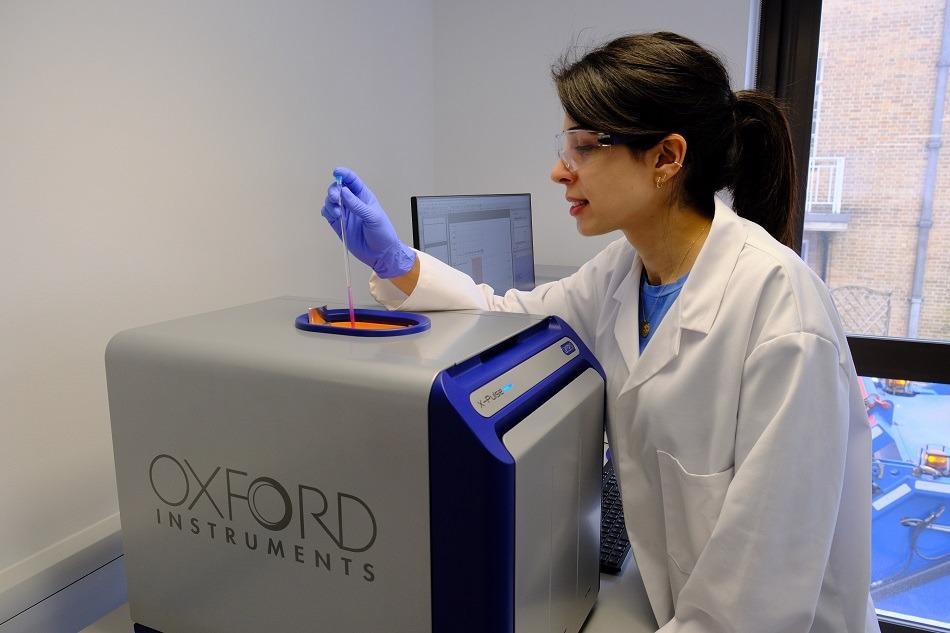
Image Credit: University of Oxford, Professor Peter Bruce Group
How is benchtop NMR employed in the analysis of battery electrolytes?
A typical battery will contain an anode, a cathode, and, between those, electrolytes and an embedded porous separator. The electrolyte contains a lithium salt, the most common being lithium hexafluorophosphate, lithium tetrafluoroborate, and lithium TFSI ((bis(trifluromethanesulfonyl)imide)). The salt is dissolved in an organic solvent, such as ethylene carbonate or dimethyl carbonate, plus additives to improve the performance of that electrolyte. These additives either enhance stability to prevent decomposition or improve the formation of a solid electrolyte interface layer at the electrodes. This passivation layer protects the electrodes while still allowing current transfer (but can also cause battery capacity loss).
When we think about what a good battery looks like, we often break it down into five key parameters:
- The energy density, for example, how much charge can be stored?
- The power density, for example, how quickly can we use that charge?
- Safety
- Costs
- The lifespan, for example, the number of charge cycles that a battery will hold its charge for.
We can better understand energy density by measuring the salt concentration in electrolytes to develop higher power density formulations. By determining the diffusion coefficients of the different species in our electrolytes, we quantify the ionic conductivity and the transference numbers of those electrolytes. These parameters and their time and temperature-dependent behavior also impact the cell’s power and energy density as well as battery lifetime.
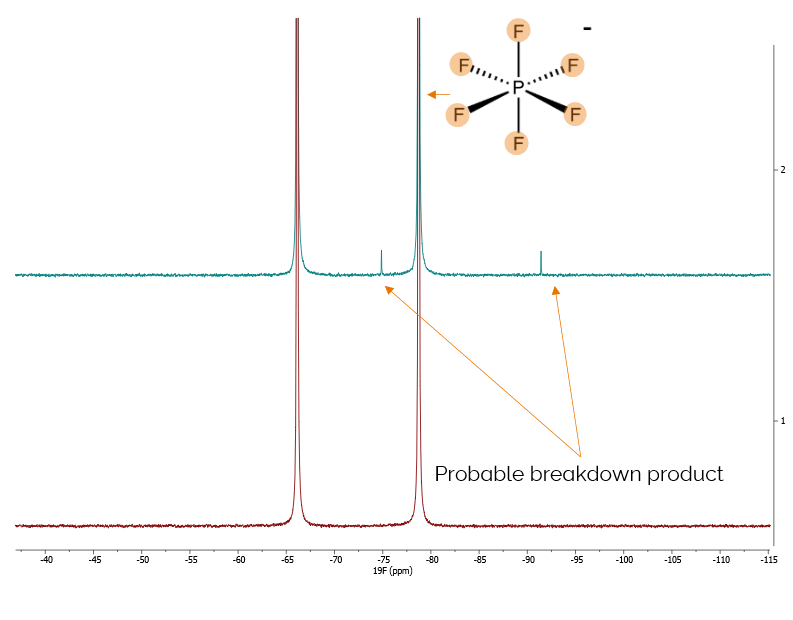
Image Credit: Oxford Instruments
Electrolyte quality is benchmarked by verifying raw materials purity, and we can also get rapid feedback on the effect of novel formulations on developing new additives and more stable electrolytes.
More specifically for lifespan, we can monitor electrolyte breakdown reactions and understand their processes. For example, trace levels of water can accelerate the formation of unwanted hydrofluoric acid (HF) based breakdown products. This process knowledge feeds back into the development of additives specifically to inhibit breakdown.
Hence, batteries can be made more cost-effectively through rapid NMR analysis of new electrolyte formulations, accelerating development, and reducing overheads. In addition, a rapid test that gives us a quantification of the quality of a final electrolyte product significantly reduces the number of defective batteries and extends battery life.
Can you give our readers a practical example of the use of benchtop NMR in quality control?
A customer wanted to help to improve their quality control processes by finding out if benchtop NMR could accurately determine their solvent composition. Specifically, can poor quality material and good quality materials be distinguished between? If this is the case, what are the causes of failure in poor-quality material?
The customer’s samples could be nominally described as lithium hexafluorophosphate and a combination of ethylene carbonate and ethyl methyl carbonate. We were supplied with two examples believed to have the same chemistry. However, the customer reported a difference in performance when these two colorless liquid electrolytes were placed in a cell.
We approached this case in the same way that most NMR spectroscopists would do when presented with a sample – we took a quick hydrogen spectrum. We could clearly identify both the ethylene carbonate and the ethyl methyl carbonate, as well as a small peak that we associated with vinylene carbonate, a typical stabilizing agent.
Once we understood which peaks were associated with which solvents, we integrated peaks to accurately measure the weight percentage contributions of both solvents.
We discovered that there was no difference between those spectra, suggesting that the solvents were chemically identical. The performance issues must be stemming from elsewhere.
Next, we took a fluorine NMR spectrum to understand the electrolyte anion coming from the hexafluorophosphate lithium salt. We could immediately see a difference in the spectrum.
We saw a doublet on the coupled spectrum arising from the coupling of the fluorines to the phosphorus in the hexafluorophosphate.
We also saw a different doublet at a different frequency, which was from a decomposition product, suggesting that the breakdown of the salt was the reason for that difference in performance.
We observed that the difference in performance is most likely the lithium salt breaking down through common hydrolysis reaction path. This analysis helped the customer to address these electrolyte performance issues, demonstrating the usefulness of benchtop NMR in quality control applications.
How common a problem is a decomposition when working with electrolytes in batteries, and how can benchtop NMR be used to address this?
Common electrolytes such as lithium hexafluorophosphate, tetraborate salts, and carbonated solvents can decompose through many different routes.
Characterizing those pathways is key to developing safer, longer-life batteries, and all of these can be monitored using benchtop NMR. To understand this, it is helpful to look at an example hydrolysis path.
Adding a single drop of water to a typical commercial electrolyte of hexafluorophosphate in dimethyl carbonate will result in a hydrolysis reaction that sees hexafluorophosphate breaking down into a combination of lithium fluoride and pentafluorophosphate, with the latter breaking down further to produce both hydrofluoric acid and fluorophosphoric acids. If enough water is present, phosphoric acid will be produced as well.
X-Pulse by Oxford Instruments
Taking spectra every 30 minutes, we track reaction progress, which is quite drastic and occurs quickly with even a relatively small amount of water.
By recording and comparing this series of spectra, we observe the reaction rates and the quantities of decomposition products forming through different stages of decomposition. Ultimately, this allows us to better manage and address these risks.
How can pulsed-field gradient NMR be used in the development of new liquid electrolytes?
Often, only ionic conductivity and the viscosity of these new electrolyte formulations are measured.
Measuring the diffusion coefficient of the different ion species, as well as the transference number (the amount of charge carried by a particular ion species), is recommended. This provides a much better prediction of the performance of an electrolyte when we put it in a cell. We can record all of these parameters using pulsed-field gradient spin echo NMR sequences. These track a change of signal amplitude proportional to the self-diffusion coefficient of a molecule.
To investigate this, we excite our sample with a typical 90-degree pulse before applying a gradient pulse across that sample to prompt a change of phase across the sample. Applying another 180-degree pulse and a further gradient pulse would allow us, if there was no diffusion, to see exactly the same signal that we had previously.
However, if molecules in our solution diffuse over the time between those two gradient pulses, we would see a decrease in the signal intensity dependent on the strength of that gradient pulse and the molecular diffusion coefficients.
After taking a series of spectra at different gradient strengths, we integrate the peaks in our spectra, and with increasing gradient strength, we should see a decrease in signal.
We fit this data to the Stejskal-Tanner equation to calculate the diffusion coefficient for the specific species in the solution. With a broadband NMR, we can measure diffusion coefficients for the solvent molecules using hydrogen, as well as each ionic species using lithium, fluorine, boron, or phosphorus. Once we have those diffusion coefficients, we can calculate the ionic conductivity of the electrolyte and the cation transference, which are highly useful parameters in the development of new electrolytes.
We can also use measurements on electrolytes at different stages of decomposition to understand how the breakdown process affects diffusion, and therefore, the physical properties of an electrolyte.
Finally, can you briefly summarize the reasons why benchtop NMR instruments like the X-Pulse are ideal for use with battery materials?
Overall, the relatively simple spectra that we get from battery materials are perfect for benchtop NMR and vice versa. We make measurements of raw materials and their quality in minutes, as well as quantifying key material concentrations.
In R&D, we get almost instantaneous feedback on new formulations to ensure that we are making what we expected to make and that we understand key factors affecting performance, such as the concentration of stabilizers and additives.
Diffusion experiments allow us to unlock the physical properties of electrolytes. In quality control, we can perform very quick measurements to determine whether any contaminants are present or whether any decomposition is occurring.
All of these experiments and investigations ultimately improve the quality, performance, and cost of the final product.
About Dr. James Sagar
James Sagar has been a Strategic Product and Applications Manager in benchtop NMR at Oxford Instruments since January 2019. He joined the company in 2015 as Product Manager for energy dispersive X-ray analysis, looking after the world’s first EDS detector for electron microscopes capable of detecting Li X-rays. Before this, James carried out post-doctoral research at University College London.
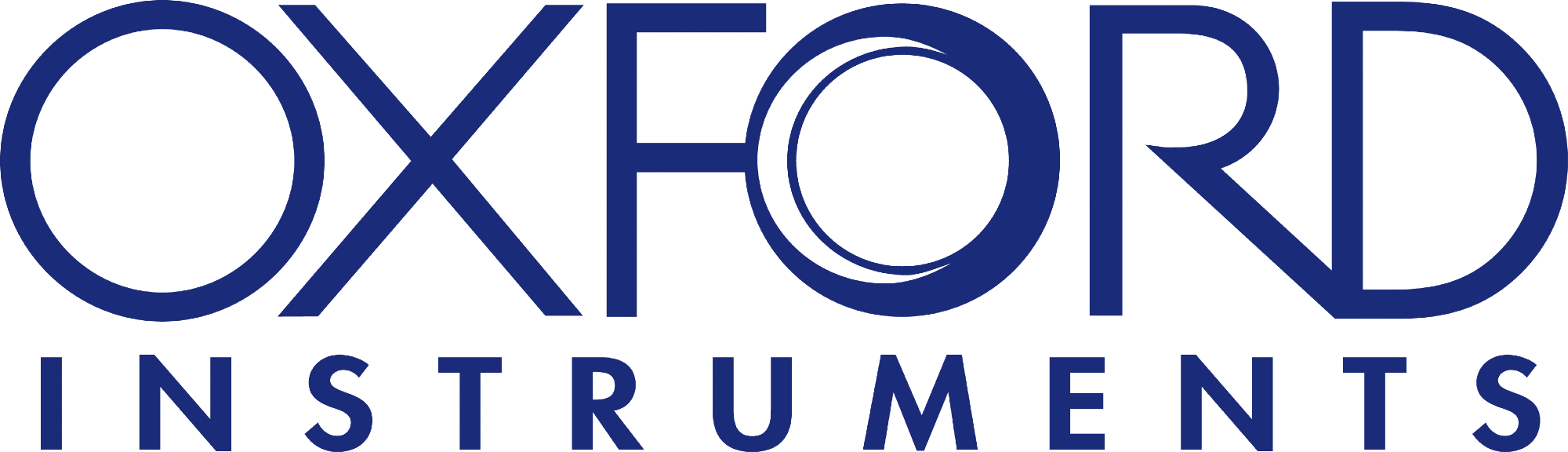
This information has been sourced, reviewed and adapted from materials provided by Oxford Instruments Magnetic Resonance.
For more information on this source, please visit Oxford Instruments Magnetic Resonance.
Disclaimer: The views expressed here are those of the interviewee and do not necessarily represent the views of AZoM.com Limited (T/A) AZoNetwork, the owner and operator of this website. This disclaimer forms part of the Terms and Conditions of use of this website.