Sponsored by MerckJan 4 2021
Carbon nanotubes are materials that have extraordinary attributes and present exceptional opportunities. This article provides a succinct summary of the physico-chemical nature and depiction of single-walled nanotubes (SWNTs). The present state of endeavors to investigate the SWNT agreement and the level of their commercialization.
Following their discovery in 1991 by Ijima1, single walled carbon nanotubes have inspired a large amount of interest in the global research community and industry and have encouraged a lot of investment in manufacturing techniques, characterization and application advancement. This is because, due to the extraordinary attributes these materials have and the variety of separate species, each possessing unique variants in those attributes.
SWNTs and MWNTs possess some parallels but also clear differences. MWNTs can be considered as a series of single walled tubes nested within each other. There may be as little as two or as much as 100 plus concentric walls. Their diameters could be as large as 50 nm compared with 0.7 - 2.0 nm for a classic SWNT. Just the outer wall usually supports the electrical and mechanical assets of MWNTs when employed in composites, for instance, providing the prospect for significantly less loading of SWNTs compared with MWNTs.
Of both of the carbon nanotube types; single walled nanotubes are the most extraordinary. They have exceptional strength, can be highly electrically conducting or semiconducting, may be as thermally conductive at room temperature as any other established material, have an extremely large surface area per unit mass and possess distinctive optical properties. This array of exceptional properties have allowed for developments in performance in a broad variety of materials and devices.
Structure of Carbon Nanotubes
Single walled carbon nanotubes are an allotrope of sp2 hybridized carbon, like fullerenes. The structure may be considered a cylindrical tube made up of 6-membered carbon rings, like in graphite. The cylindrical tubes can possess one or both ends capped with a hemisphere of the buckyball or fullerene structure.
A comprehension of SWNT structure needs experience with the idea of nanotube chirality because the chirality of an SWNT determines a lot of its properties. A concept identified as a Chirality Map, shown in Figure 1, has been cultivated as a tool for comprehending chirality and its consequences.
An SWNT can be envisaged as a sheet of graphite one atom thick rolled into a tube (see inset in Figure 1). The chirality illustrates both the orientation and diameter to which the sheet is rolled. Every SWNT on the chirality map is characterized by two integers, (n,m). Chirality identifies a lot of the properties of the individual SWNT. For instance, the SWNT displayed on the chirality map in blue is metallic in nature. These are tubes where n=m (armchair) or n - m = 3i (where i is any integer.) Those shown in yellow are semiconducting, exhibiting different band gaps varying on the length of the chiral vector.
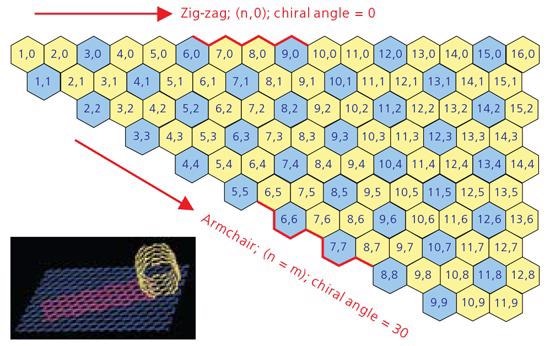
Figure 1. A graphic displaying a Chirality Map which shows the various types of SWNTs that can be forfmed.The properties are governed by the way in which they are rolled as shown in the insert. The SWNT will be metallic in the armchair configuration, or when m-n is a multiple of 3. Image Credit: Merck
Distinct Properties of Single Walled Carbon Nanotubes
Mechanical
Individual SWNTs are substantially tougher than steel. Determined values for tensile strengths of SWNTs are ~100 times larger than steel at 1/16th the weight. The maximum measured value is roughly half of the projected theoretical strength,2 perhaps because of flaws in the structure.
Electrical
Individual SWNTs, at present, possess carrying capabilities of 109 amp/cm2, bigger than those of copper or gold,3 and semiconducting species display larger electron mobility than silicon.
Optical
SWNTs have a distinctive optical absorption and fluorescence reaction, with every chirality exhibiting its own characteristic absorption and fluorescence spectrum. Broadly, coating created with SWNTs is extremely transparent in the detectable and IR areas of the spectrum, meaning SWNTs are an optimum candidate to substitute ITO because of the transparent conductor of choice for applications like displays, solar cells and electroluminescent lighting etc.
Thermal
Room temperature thermal conductivity of one nanotube can be compared with that of diamond or in-plane graphite, which are usually considered to show the most measured thermal conductivity of any recognized material at mild temperatures.
The Challenges of Single Walled Carbon Nanotubes (SWNTs)
Technical obstacles in the fields of purity, selectivity and dispersibility have as yet restricted the extensive use of SWNTs. A lot of recent progress has occurred to tackle all of these barriers.
Purity
The numerous manufacturing methods employed in the manufacture of SWNTs results in products which are tainted to be different degrees with residual catalyst and additional forms of carbon. For a lot of applications, secondary processes are required to eliminate these contaminants to deliver a product of adequate purity. More recently, techniques of synthesis that lessen the ‘as manufactured’ contaminations have grown increasingly commercially.
Selectivity
The SWNTs are a combination of tubes of varying chiralities, some of which are electrically conducting and some are semiconducting. It is attractive, for a lot of applications, to separate the kinds of tubes from one another, like metallic from semiconducting and for certain applications, tubes with well-defined individual chiralities (see previous section for an account of SWNT chirality). Laboratory scale techniques intended to accomplish an extremely high degree of selectivity have been reported,4 and attempts to create scalable processes are in progress. Manufacturing practices like the CoMoCAT® catalytic CVD process have shown the ability to offer a significant degree of selectivity toward specific chiralities in as-synthesized SWNTs, meaning the yield of secondary purification processes is significantly increased.
Dispersibility
SWNTs can be challenging to diffuse, in part due to their well-known inclination to create ropes or bundles because of natural Van der Waals attraction across the tubes. Despite this, they may be distributed in aqueous solutions with the help of appropriate surfactants either as small bundles or as individual tubes.
Exfoliation of bundles may be accomplished by sonication of aqueous solutions of SWNTs in the presence of surface-active molecules like DNA, sodium deoxycholate (Prod. No. D6750) and sodium cholate. To calculate the degree of nanotube exfoliation achieved in each dispersion, Tan and Resasco5 characterized the notion of resonance ratio from the optical absorbance spectrum. The vicinity of the resonant band separated by the area of the non-resonant background in this ratio permits simple contrast of outcomes separate from the complete absorption. Dispersants can be ranked following this for their efficiency employing this parameter.
Dispersions of SWNTs in resins and thermoplastics is restricted by a remarkable increase in viscosity triggered by the entanglement of the SWNT bundles. Numerous proprietary techniques occur to circumvent this issue and new hybrid elements of SWNTs are being created to tackle this problem.
SWNT Synthesis
Numerous techniques have been employed in the production of SWNT. These consist of Laser Ablation, Carbon Arc and CVD processes, either involving a gaseous catalyst as in the HiPCo® process, or employing a supported catalyst as in the CoMoCAT® process. The laser ablation method is employed principally for investigation materials. The Carbon Arc process creates long tubes with diameters in the range of 1.4 to 2.0nm, but carbon arc material has an extremely large amount of impurity and, for many applications, will need extensive purification. The CVD processes provide the optimum attitude to the manufacturing of larger SWNT quantities, with possibly the most scalable being the CoMoCAT® process, which employs a fluidized bed reactor (Figure 2) like those employed in petroleum refining, although on a significantly smaller scale.
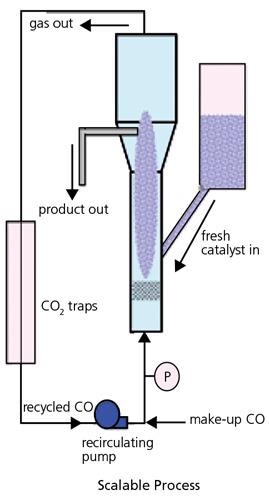
Figure 2. An illustration of a fluidized bed reactor which is able to scale up the generation of SWNTs using the CoMoCAT® process. Image Credit: Merck
In this CoMoCAT® technique, SWNT are grown by CO disproportionation (decomposition into C and CO2) at 700-950 ºC in flow of pure CO at a complete pressure that generally ranges from 1 to 10 atm. In a three-year research program of catalyst and reactor expansion, which consisted of comprehensive characterization and testing of numerous catalyst formulations and operating conditions, a process that can grow substantial quantities of SWNT in under one hour was developed, maintaining a selectivity towards SWNT better than 90 percent. A synergistic effect between Co and Mo that is vital for the performance of the catalyst was discovered. The catalyst is efficient when both metals are present at the same time on a silica support with a low Co:Mo: separated; they are unselective. Figure 3 displays the selective synthesis of an SWNT applying the CoMoCAT® technique.
Two of the distinct characteristics of the CoMoCAT® process are that it is quickly scalable and its inherent high selectivity is protected as the reactor size is scaled up. These characteristics convey the SWNT product of the CoMoCAT® process the dual benefit of cost-effective and high product quality. This supported catalyst approach also provides the distinctive capacity to offer a significant degree of chirality control throughout synthesis.
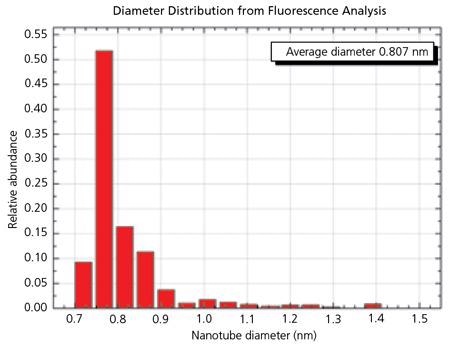
Figure 3. The Histogram for SG 65 material shows the very narrow distribution of SWNT diameters possible with the CoMoCAT® process. 90% of the tubes have a diameter between 0.72 and 0.92 nm. 52% of the tubes are (6,5) chirality. Image Credit: Merck
Characterization of SWNTs and Quality Assurance Parameters
The properties of carbon nanotubes differ with the individual SWNT chirality. Because at this time, all SWNTs are generated as a combination of chiralities, the aspects of the material will hinge on the quantities of chiralities present. A lot of analytical methods have been implemented to elucidate the structure of SWNT materials. These vary from observational techniques like SEM, TEM, AFM and STM to spectroscopic techniques such as UV-Vis- NIR, Photoluminescence and Raman spectroscopy.
As well as these techniques, X-ray diffraction has been employed by Miyata et al. 6 to corroborate the chirality assignments in the optical spectrum of SWNTs. Thermogravimetric analysis (TGA) has been employed widely to ascertain the onset of oxidation, maximum oxidation rate and the mass of catalyst preserved in the product. In some instances, it is feasible to obtain a realistic assessment of purity from the TGA curve.
TEM and SEM have been broadly employed to evaluate SWNT purity. Despite this, these are inaccurate for any quantitative estimation of purity. A standard TEM or SEM image uses ~1 pg of material over an area of 1 to 4 μm2 and as such, it would take the analysis of a lot of micrographs imaged at random through a macroscopic sample to achieve any significant outcomes of the total purity.
There are no appropriate algorithms for accurately verifying the comparative proportions of the various species seen in standard unpurified SWNT material. As such, while TEM and SEM can provide relevant information on the structure of the product, they need to be employed with vigilance and deemed only as qualitative signs of purity.
There are three reasonably simple and universally accessible methods that may be employed in combination to guarantee that reliable high quality SWNTs are manufactured; they are Raman spectroscopy, Absorbance spectroscopy and Thermogravimetric analysis (TGA). Collectively, these three techniques provide an appropriate measure of purity and stability of the SWNT.
Despite this, as SWNT applications are established more, functional testing, like electrical conductivity measurements, will be required to connect the purity data to SWNT performance.
Analysis by Raman Spectroscopy
Raman spectroscopy has been broadly employed for ascertaining both the detailed mixture of chiralities present in the SWNT material and for evaluating purity. There are three sections of the Raman spectrum of primary interest for SWNTs. The Radial Breath Mode (RBM) from roughly 120 to 300 cm-1 is distinctive to SWNTs and can be employed to ascertain tube diameter from the equation:
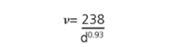
where, d is the SWNT diameter in nm and ν is the wavenumber in cm-1.
It is critical to note that to gain a whole picture of the chiralities present, many lasers of various excitation frequency must be employed. Applying a constantly variable laser to excite the SWNT, Jorio et al. have charted the chiral structure of SWeNT® SG 65.7
There are two further bands present in the Raman spectrum of SWNT: The D band at 1300 to 1350 cm-1 suggests disordered carbon, multiwall tubes and microcrystalline graphite and the G band at 1500 to 1586 cm is a consequence of the tangential stretching mode from graphitic-like materials. The ratio of the height of the G band to that of the D band has been broadly employed as a measure of the integrity of SWNT.
Despite this, caution must be employed when measuring this ratio as the G band is a resonant band and, as such, is significantly tougher than the D band. It is likely to be best used by asserting that a high G:D ratio is a requirement for high purity SWNT, but it is an inadequate guarantee of purity as other techniques must be employed in conjunction with this parameter. For instance, other forms of graphitic carbon might be a factor of a strong G band.
The Raman G:D ratio, with the cautions, may be employed as a first measure of purity. A standard Raman Spectrum for SWeNT® SG 65 can be seen in Figure 4. For quality assurance objectives, the RBM region can be employed as a general purity fingerprint.
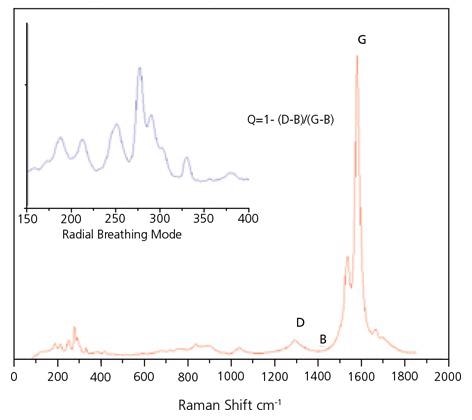
Figure 4. Typical Raman spectrum of SWeNT® SG 65, obtained with 633 nm laser excitation. Image Credit: Merck
Analysis by Optical Absorbance Spectroscopy
Optical absorption (OA) measurements in the UV-Vis.-NIR region display peaks which are typical of individual (n,m) species superimposed on the π-plasmon background. For instance,8-9 the (6,5) species absorb at 566 and 976 nm and, in reaction, fluoresce at 983 nm. A (7,6) SWNT absorbs at 645 and 1024 nm and fluoresces in response at 1030 nm.
These specific peaks have been employed as a foundation for assessing the purity of SWNT.10 Nair et al.11 have created a technique for computing the baseline for the spectrum, which then allows for a calculation of peak heights and regions for the individual (n,m) species. For ease, the measured OA spectrum is normally transformed to the energy domain, where the background becomes linear in attention for SWNT characterization.
Figure 5 displays a standard OA spectrum for SWeNT® SG 65. The inset depicts the spectrum in the additional conventional form with the absorption plotted as a function of wavelength, while Figure 5b displays the same spectrum transferred to the energy domain. Measurements of the height of the strongest peak (P2B) and incorporation of the overall signal, S2B, can be employed to ensure that the product is reliable. P2B is primarily used as a control parameter for SWeNT® SG 65 and SG 76 nanotubes, where one specific tube type is dominant. P2B is characterized as the height of the highest peak in the spectrum between 350 and 1,350 nm separated by the background at that wavelength
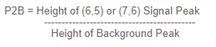
It should be noted that the OA methodology, as described here, employs the OA spectrum measured following the dispersing and centrifuging of the SWNT sample. It is employed as a measure of chirality control instead of overall purity. Measurement of the absorbance at a specific wavelength prior to and following centrifugation provides a measure of the dispersibility of the SWNTs.
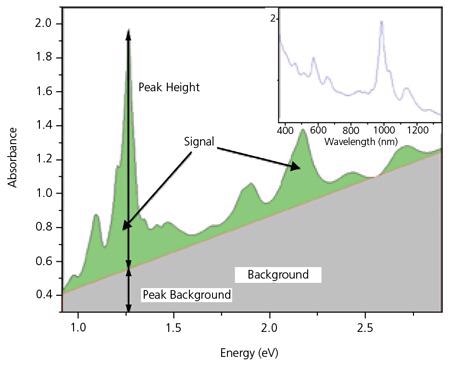
Figure 5. Optical Absorbance spectrum for SWeNT® SG 65. The highest peak corresponds to the (6,5) tubes. Image Credit: Merck
Thermogravimetric Analysis
Thermogravimetric analysis (TGA) is used to evaluate the purity of the material. A standard TGA curve for SG 65 SWNT can be seen in Figure 6. Studies have shown that the initial peak in the derivative curve of the TGA trace signifies the oxidation of SWNT, while the second peak is indicative of the presence of additional forms of carbon. The quality parameters established from the TGA analysis are T1% and residual mass at 625 °C.
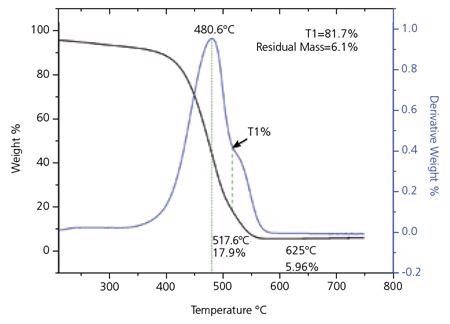
Figure 6. Thermogravimetric (TGA) Analysis for SWeNT® SG 65. The small peak in the derivative curve about 625 °C is due to changes in the residual catalyst as the material is heated. Image Credit: Merck
T1%, seen in Figure 6, is measured as a control parameter. It has been demonstrated that this measurement usually underestimates the SWNT content by 3-5%. The position for T1 is taken at the least between the two peaks in the derivative curve. In the absence of a second separate peak in the derivative curve, T1 is taken at the point of inflection. The weight loss % is recorded and the final value of T1% as a percentage of the carbon in the sample, adjusted for the preliminary weight loss because of moisture in the sample, is determined from the following equation:
T1% = Initial weight - T1% measured
------------------------------------------
Initial weight - residual mass
The measurement of residual mass at 625 °C gives a measure of the non-carbon content of the material. The residual mass is expressed as a percentage normalized for the weight loss at 200 °C.
Residual Mass = Weight Loss at 625 °C
-------------------------------
Initial Weight Loss
SWNT Applications
The many unique properties of SWNTs have led to their application in a variety of technological issues.12 Their remarkable mechanical fortitude is utilized in enhanced carbon fiber13 and reinforced resins and elastomers; their extremely conductive nature and large surface areas are used to prepare conductive polymer blends and films, enhanced lithium-ion batteries and supercapacitors. Unique optical properties mean they can be used as electrodes in displays, solar cells and developing solid-state lighting technologies. The semiconducting nature of some SWNT species means they can adapt to logic devices, non-volatile memory elements, sensors and security tags. New SWNT applications appear regularly, restricted only by the creativity of scientists and engineers working in the field.
Conclusion
In spite of early enthusiasm about SWNT materials and the remarkable level of research encouraged by their discovery, to date, commercial exploitation of the technology has been disheartening. This could be because there is insufficient comprehension of the practical hurdles to their commercialization. However, momentum now seems to be building, driven by significant recent progress in these key areas:
Metrology and Quality Control: The notion of “if you can measure it, you can improve it” applies here. The means are now accessible to sufficiently characterize SWNTs and to guarantee consistency of the materials required for commercialization. Endorsing this is the soon to be accessible offerings by NIST of Standard Reference Materials for calibration purposes.
Improved selectivity: Driven by applications that need more than a near-random allocation of tube chiralities, there has been a presentation of the means to significantly narrow the ‘as produced’ chirality distributions of commercial scale production products. There is also encouraging work toward accomplishing further selectivity through secondary processing.
Dispersion: Recent years have seen the advent of enhanced aids to disperse SWNTs for formulation in inks and composites.
Scale-up of manufacturing process: In the last five years, there has been an advancement and a maturing of scalable SWNT manufacturing processes, which can offer commercial quantities of SWNT with high purity, regulated properties and reliable quality.
References and Further Reading
- Iijima, S. Nature, 1991, 354, 56.
- Meo, M.; Rossi, M. Composite Science and Technology, 2006, 66,1597.
- Tans, S.J.; Devoret, H.; Thess, A.; Smalley, R.E.; Geerligs, L.J.; Dekker, C. Nature, 1997, 386, 474.
- Arnold, M.S.; Green, A.A.; Hulvat, J.F.; Stupp, S.I.; Hersham, M.C. Nature Nanotechnology, 2006, 1, 60.
- Tan, Y.; Resasco, D.E. J. Phys. Chem. B, 2005, 109, 14454.
- Miyata, Y.; Yanagi, K.; Maniwa, Y.; Tanaka, T.; Kataura, H. J. Phys. Chem. C, 2008, 112, 15997.
- Jorio, A.; Santos, A.P.; Ribeiro, H.B.; Fantini, C.; Souza, M.; Viera, P.M.; Furtado, C.A.; Jiang, J.; Balzano, L.; Resasco, D.E.; Pimenta, M.A. Phys. Rev. B, 2005, 72, 075207.
- Bachilo, S.M.; Strano, M.S.; Kitrell, C.; Hauge, R.H.; Smalley, R.E.; Weisman, R.B. Science, 2002, 298, 2361.
- Lolli, G.; Zhang, L.; Balzano, L.; Sakulchaicharoen, N.; Tan, Y.; Resasco, D.E. J. Phys. Chem. B, 2006, 110, 2108.
- (Itkis, M.E.; Perea, D.E.; Jung, R.; Niyogi, S.; Haddon, R.C. J. Amer. Chem. Soc., 2005, 127, 3439.
- Nair, N.; Usrey, M.; Kim, W-J.; Braatz, R.D.; Strano, M.S. Anal. Chem., 2006, 78, 7589.
- Rensselar Polytechnic Institute. Ajayan and Zhou.
- Jorio, A.; Dresselhaus, G.; Dresselhaus, M.S. (Eds.). Carbon Nanotubes, Topics in Applied Physics.,: Springer-Verlag, New York (2008).
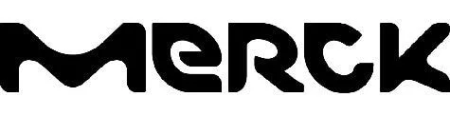
This information has been sourced, reviewed and adapted from materials provided by Merck.
For more information on this source, please visit Merck.