The combination of catalysis and plasmas under moderate temperatures is an emerging field [1]. There are two ways in which techniques are usually combined. The first is by introducing a catalyst in the plasma discharge (in plasma catalysis, IPC), and the second is by positioning the catalyst after the discharge zone (post plasma catalysis, PPC). The introduction of plasma into a catalytic system might have several outcomes, for instance, a change in catalyst structure, a change in catalyst properties such as an increase in dispersion, or a change in the type or distribution of reactive species available for reaction.
A microreactor that enables the investigation of catalysis using conventional temperature programed techniques has been developed. However, the reactor also allows the generation of a dielectric barrier discharge (DBD) over the entire length of the catalyst region (IPC). DBD produces cool atmospheric plasma and is a standard technique for generating surface modifications and as a source of radicals and ions for reaction processes. Several test reactions have been analyzed to show the difference in activation temperatures and reaction product distributions when compared with the catalyst alone. Measurements of reaction product distributions were carried out using a traditional capillary inlet mass spectrometer. The reactive gas species produced inside the plasma/catalyst system was sampled using a molecular beam inlet mass spectrometer (MBMS). These two techniques, when combined, give a unique insight into plasma catalysis process.
Atmospheric-Pressure Plasma Reactors
It is possible to operate various electrical plasmas at atmospheric pressure. The range of application of such plasmas in fields such as chemical synthesis, surface modification, and waste gas treatment is growing rapidly. Versatile examples are plasma sources designed around discharges produced over the surface of a dielectric covering one of the discharge electrodes (“dielectric surface barrier discharges”). Generally, the plasma is acquired using a high-frequency source to produce a discharge in a flow of helium to which two or more reactant gases are added. The plasma is made up of ionized, excited, and near-thermal gas species and also electrons with energies of up to 25 eV.
Experiment
A microreactor based on the Hiden CATLAB was built to enable a catalyst to be heated under controllable gas flow and temperature conditions. The microreactor was connected to the Hiden HPR-60 molecular beam mass spectrometer system (MBMS). The MBMS enables analysis of the gaseous species as well as the ions and radicals produced in the plasma. Apart from the standard furnace arrangement, a dielectric barrier discharge (DBD) could also be produced over the length of the catalyst or either before or after the catalyst. The DBD included an outer cylindrical metallic electrode wrapped around the quartz tube and connected to ground, and an inner coaxial tungsten wire electrode of 1.0 mm diameter. The tungsten electrode is connected to the open-circuit end of the secondary winding of a high voltage transformer operated at 50 kHz [4].
Reactions were carried out using 1% Pd/Al2O3 catalyst. Oxidation of CO to form CO2 was the test reaction used. It was observed that in all the experiments, the concentration of O2 exceeded the amount needed to entirely convert CO. Figure 1 shows a schematic of the experimental setup.
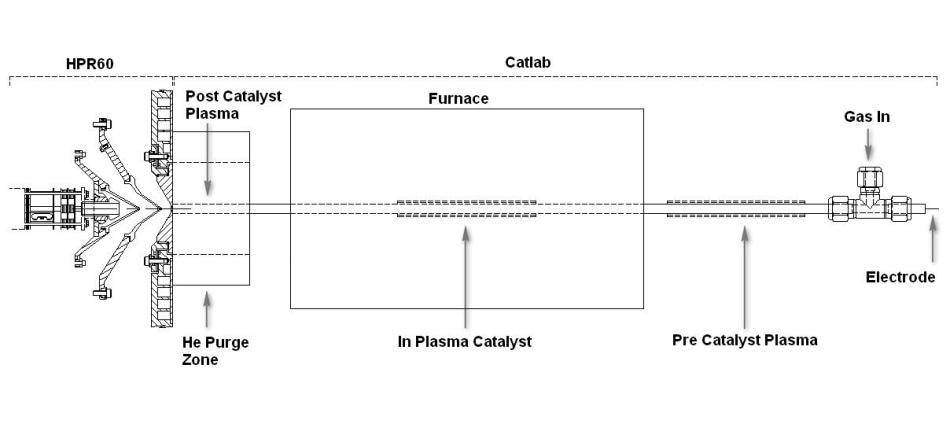
Figure 1.
Results
In the blank experiments (no plasma, no catalyst, not shown), it was observed that there was no reaction at temperatures under 500 °C. The results of using the plasma alone for the oxidization of CO are shown in Figure 2. The figure shows that some conversion of CO to CO2 took place immediately once the plasma was switched on.
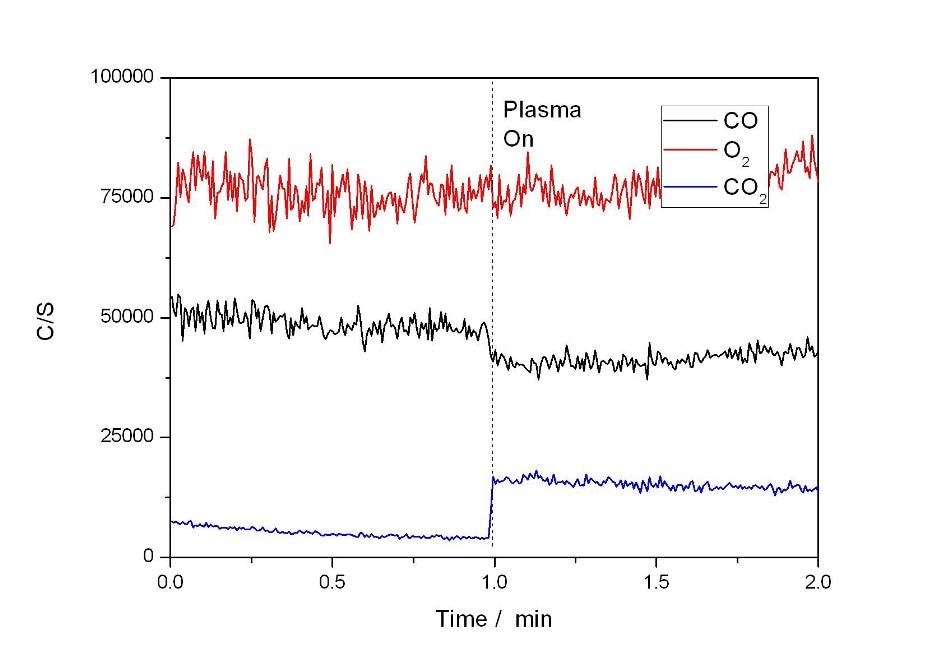
Figure 2.
Figure 3 shows the results of using the catalyst alone. The sample was ramped at 15 °C/min to 600 °C. The figure shows that total conversion of CO to CO2 took place at approximately 250 °C.
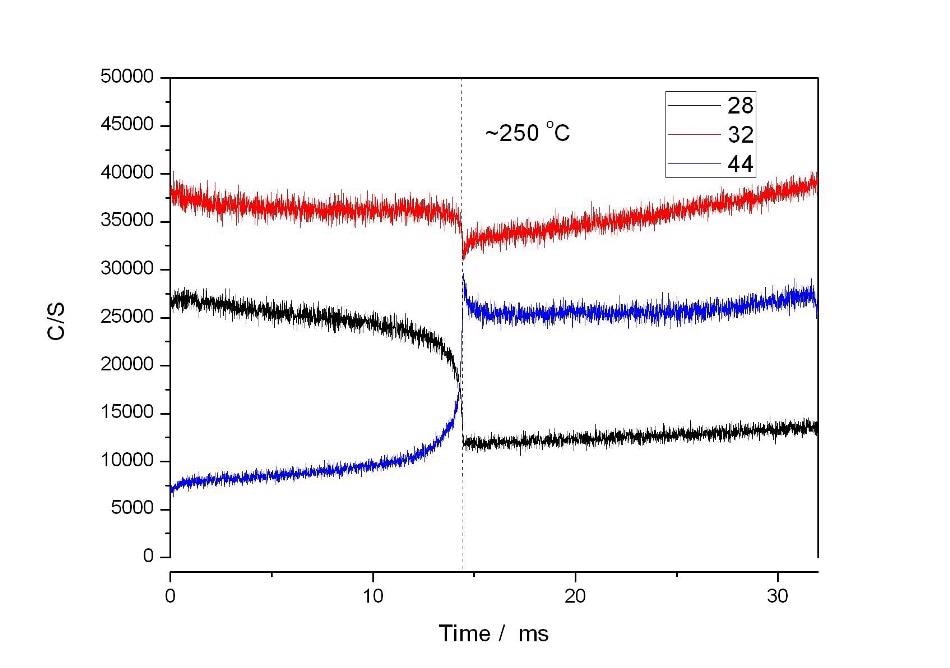
Figure 3.
Figure 4 shows a plot which is the result of the combined plasma and heating experiment. Here, the plasma was generated over the length of the catalyst. The plasma was switched on before the heating was initiated. At this point, an initial increase in CO2 production can be observed. Upon heating, it was observed that CO was entirely converted to CO2 at 150 °C, 100 °C below the temperature at which reaction occurs with the catalyst and temperature alone.
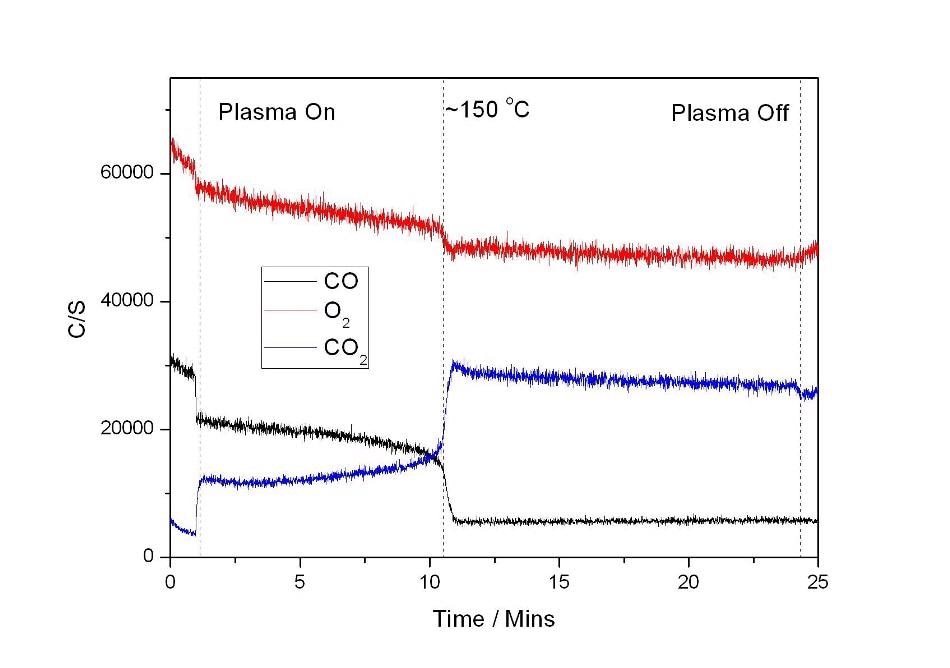
Figure 4.
The plot depicted in Figure 5 is the result of the combined plasma and catalyst heating experiment. Here, the plasma was generated in a region before the catalyst bed. Prior to heating, the plasma was switched on and showed the same initial increase in CO2 production as before. Upon heating, complete conversion of CO to CO2 was observed at 150 °C. The result of the experiment is identical with the plasma generated over the length of the catalyst. This indicates that the decrease in temperature observed in the reaction going to completion is the result of the ionized gas species created in the plasma being more reactive over the catalyst and not the result of any change to the catalyst surface by the plasma being responsible for the decrease in reaction temperature.
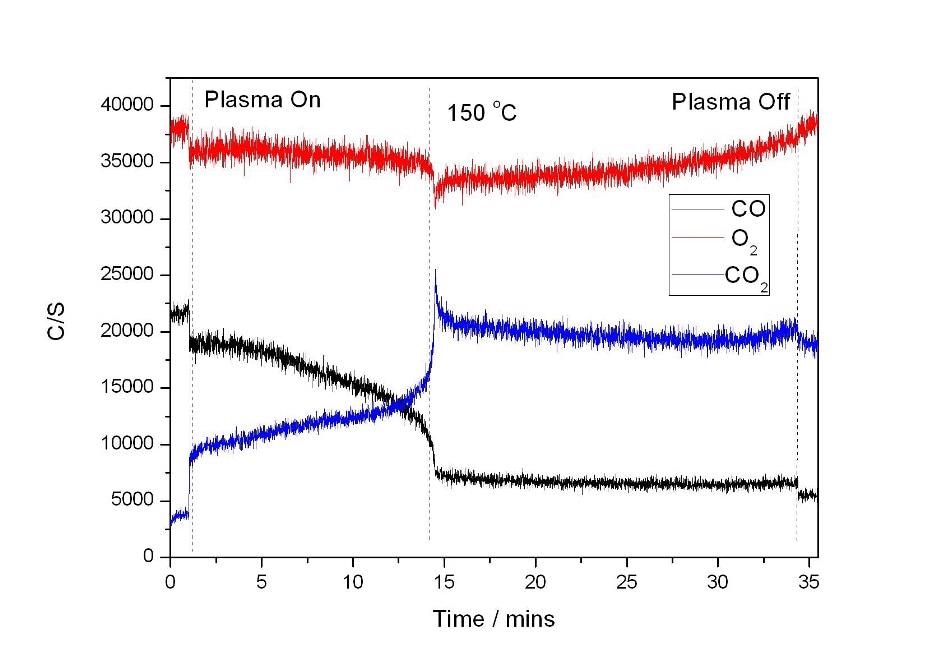
Figure 5.
Conclusions
In the model reactor described in this article, oxygen and carbon monoxide are used as the reactant gases. The reactive species produced in the plasma include atomic oxygen and ions such as CO+, O+, O2+, CO2+, O-, O2-, and CO3-. These species, on interaction, produce carbon dioxide as the major reactor product. The conversion of CO into CO2 using IPC is clearly of interest, just as potential enhancements in the efficiency of the process through control of the plasma conditions. The overall efficiency of the conversion can be significantly increased by exploiting the synergy between catalysts and plasmas.
References
[1] J.Van Durme, J. Dewulf, C. Leys, H. Van Langenhove, Appl. Catal. B Environ. 78 (2008) 324–333.
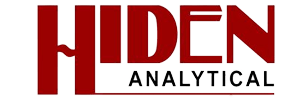
This information has been sourced, reviewed and adapted from materials provided by Hiden Analytical.
For more information on this source, please visit Hiden Analytical.