Sponsored by HORIBAJun 14 2018
In X-ray fluorescence (XRF) spectroscopy, the interaction of X-rays with a material is used to determine its elemental composition. It can be used for the analysis of solids, liquids, and powders, and is generally non-destructive. There are two main XRF methodologies - energy dispersive (EDXRF) and wavelength dispersive (WDXRF). They each have advantages and disadvantages, and mainly differ in the way fluorescent X-rays are detected and analyzed.
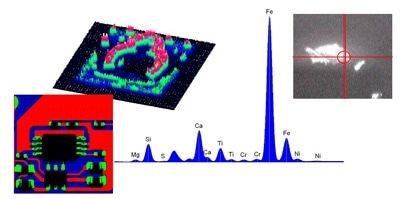
Generally, EDXRF can measure all elements from sodium to uranium and WDXRF up to beryllium, at concentrations ranging from 100% down to ppm and even sub-ppm levels in some cases. Limits of detection are dependent on the element and the sample, but heavier elements usually have better detection limits.
XRF is widely used as a fast characterization tool in applications such as metallurgy, forensics, polymers, electronics, archaeology, environmental analysis, geology, and mining. Recent advances in X-ray technology have led to XRF instruments with high spatial resolution.
X-Rays
X-rays are part of the electromagnetic spectrum and their energies lie between those of ultra-violet and gamma radiation with wavelengths between 0.01 and 10 nm (125 keV to 0.125 keV).
The properties of X-rays were first reported by Wilhelm Röntgen in 1895, and his work in this field later gained him the Nobel Prize, with the technology still named after him in a number of languages.
X-rays are widely used for medical imaging, security screening, and many elemental and structural analysis techniques.
Interaction of X-Rays with Matter
Röntgen’s studies showed that X-rays could penetrate matter, and their usefulness in medical imaging was quickly realized. However, during interaction with matter, some part of the X-rays is also absorbed or scattered.
When absorption occurs, the atomic level interaction of X-rays may also cause fluorescence, which forms the basis for XRF spectroscopy. Scattering can occur with or without loss of energy, known as Compton or Rayleigh scattering, respectively. All these interactions depend on the sample thickness, density, composition, and the energy of the X-ray.
X-Ray Fluorescence - The Basic Process
X-ray fluorescence can be considered using a 3-step process:
- An incoming X-ray knocks out an electron from one orbital that surrounds the nucleus within an atom of the material.
- This results in a high-energy unstable state because of the formation of a hole.
- For the atom to go back to equilibrium, an electron from a higher energy outer orbital falls into the hole. Since this position is of lower energy, the excess energy is emitted in the form of fluorescence.
The difference in the energy between the expelled and replaced electron is characteristic of the element. Thus, the energy of the emitted fluorescent X-rays is directly related to a specific element. This makes XRF a rapid tool for determining elemental composition.
In general, the energy of the emitted X-ray for an element is independent of its chemical composition. For example, a calcium peak obtained from CaCO3, CaO and CaCl2 will hold an identical spectral position for all three materials.
X-Ray Fluorescence - Multiple Transitions
Since most atoms have a number of electron orbitals (e.g., K-, L-, M-shells), several fluorescent transitions are possible.
For example, interaction of X-Rays with an atom with K, L and M shells could result in a hole forming in the K shell, which is then filled by an electron from the L shell or from the M shell. This is called K transition. If a hole is formed in the L shell and is filled by an electron from the M shell, it is called an L transition.
Hence, for a single element, there will be several peaks. All will be present in the spectrum at varying intensities, and together they form a “fingerprint” of the element.
X-Ray Fluorescence – Intensity
How much is absorbed by a material depends on the energy of the X-rays, and usually low energy X-rays are more readily absorbed by a material.
To knock out an electron from one of the orbitals, the X-ray energy must be more than that of the electron. However, when the X-ray energy is overly high, the coupling between the X-rays and the electron is inefficient, and only a few electrons will be expelled.
When the energy of the X-rays is similar to the binding energy, more electrons are expelled. Just below the binding energy, a drop in absorption is observed, since the energy is not adequate to emit electrons from that shell, and is too high to emit electrons from the lower energy shells.
In addition, not all X-rays result in fluorescence. The fluorescence yield is the ratio of fluorescence X-rays to incident X-rays.
XRF Spectroscopy
The key components of a typical XRF are:
- Source of X-Rays used to irradiate the sample
- Sample
- Detection of the emitted fluorescent X-Rays
The resulting XRF spectrum shows the intensity of X-Rays (usually in counts per second) as a function of energy (usually in eV).
Energy Dispersive XRF
An energy dispersive detection system directly measures the energies of the X-rays emitted from the sample. The XRF spectrum is generated by counting and plotting the relative numbers of X-rays at each energy.
The principle of the energy dispersive (ED) detector (such as the HORIBA Xerophy™) is based on the generation of electron-hole pairs in a semiconductor material (often silicon). An incident X-ray, of energy EX, is absorbed by the detector, and will instigate the formation of one or more electron-hole pairs. The energy, EEHP, to do this is fixed for that particular material. The X-ray will form as many electron-hole pairs as its energy will allow: number of electron-hole pairs = EX/ EEHP
Once this happens, electrons are pulled off the detector, and the resulting current is proportional to the number of electron-hole pairs, which is directly linked to the X-ray energy.
This analysis procedure is repeated at a very high rate, and the results are organized into energy channels.
Wavelength Dispersive XRF
In this system, the X-rays are diffracted to a crystal, which diffracts and physically separates the X-rays according to their wavelengths.
In a sequential system, the detector is stationary and the crystal rotates to allow different wavelengths to be detected, with the XRF spectrum then built point-by-point. In a simultaneous system, several crystals/detectors are used, so a range of elements can be detected at the same time.
The main difference between ED and WDXRF is in the achievable spectral resolution. WDXRF systems can provide resolutions between 5 and 20 eV, whereas EDXRF systems provide resolutions between 150 to 300 eV or more, depending on the variety of detector used.
The advantages of a higher resolution WDXRF are reduced spectral overlaps, so multifaceted samples can be characterized more accurately and high resolution backgrounds reduced, leading to better detection limits and sensitivity.
However, the WDXRF has additional optical components (e.g., diffracting crystal and collimators) causing reduced efficiency. High-power X-rays compensate for this, but can have a great impact on cost and ease of use. Along with the extra optical components, WDXRF becomes a relatively expensive instrument.
Another difference is in how the spectra are acquired. With an EDXRF system such as one of the XGT systems, an entire spectrum is acquired almost simultaneously, so that elements from across most of the periodic table can be detected within a few seconds. Using WDXRF, the spectra are acquired point-by-point, which takes a long time, or has a limited number of simultaneous detectors (an expensive option).
Micro XRF
XRF has conventionally been a bulk technique, and analysis regions range from a few millimeters to a few centimeters. Samples that are not uniform are ground and made into pellets or fused with a glass matrix, making for long sample preparation times and requiring a lot of material.
Advances in X-ray optics have led to narrow beam X-rays ranging from 1 mm to 10 µm. This allows single microscopic particles to be analyzed discretely, and detailed element images can be built with high spatial resolution.
Typically micro-XRF systems like the XGT series are based on energy dispersive detection, as generating a detailed image with thousands of individual pixels requires quick acquisition of a spectrum at each pixel position. The time involved in scanning using a WDXRF spectrometer is not viable for the imaging micro-XRF samples.
Micro-XRF is becoming popular in a wide variety of fields such as materials, geology and mineralogy, engine wear debris, gemology, archaeology, electronics, environmental science, pharmaceutics, biology, and medicine.

This information has been sourced, reviewed and adapted from materials provided by HORIBA.
For more information on this source, please visit HORIBA.