DOI :
10.2240/azojomo0279
Apr 30 2009
Ramos-Galván C., Sandoval-Robles G., Robles-Andrade S., García-Alamilla R., Llanos-Serrano M. E., Navarrete-Bolaños J. and Domínguez-Esquivel J. M.
Copyright AD-TECH; licensee AZoM.com Pty Ltd.
This is an AZo Open Access Rewards System (AZo-OARS) article distributed under the terms of the AZo–OARS https://www.azom.com/oars.asp which permits unrestricted use provided the original work is properly cited but is limited to non-commercial distribution and reproduction.
AZojomo (ISSN 1833-122X) Volume 5 April 2009
Topics covered
Abstract
Keywords
Introduction
Experimental
Results and Discussion
Conclusions
References
Contact Details
Abstract
A study was carried out on the preparation and characterization of polyacrylonitrile (PAN) combined with two silica support types, its catalytic activity being evaluated in the oxidative dehydrogenation (ODH) of ethylbenzene (EB) at 400°C and W/F 177 g cat/mol/h. The main purpose was to combine the textural properties and the thermal stability of the silica with the surface functional groups of the PAN carbonaceous phase. The material supported on the low surface area silica (SiO2 B) presented better textural properties and a higher thermal stability and catalytic activity with respect to solid supported on SiO2 A and unsupported PAN. Thus, the support type and redox/acid-base properties of PAN/SiO2 systems play an important role on their catalytic properties. The active sites seem to be the basic nature surface groups such as carbonyl/quinone, carboxylate and pyridine like groups, which act in a concerted way with the acid sites (oxhydrile-phenolic groups).
Keywords
Pyrolyzed, Polyacrylonitrile, Ethylbenzene, Oxidative, Dehydrogenation
Introduction
Pyrolyzed polyacrylonitrile (PAN) is a semiconductive material and an active catalyst in dehydrogenation reactions [1-4], due to the pyridine type aromatic structures formed during the organic precursor (PAN) pyrolysis, which acts through a redox mechanism similar to quinone/hydroquinone [5, 6]. It is well known that the nitrogen incorporation to carbons drastically increases their catalytic activity, especially in selective oxidation reactions. It is always mentioned that the carbons having an oxygen low content show basic surface properties, which are attributed to the presence of basic surface oxides; however, it has been shown that the π electron system of the carbon basal planes is sufficiently basic to bond protons from acid solutions [7]. The catalytic activity of these carbonaceous materials is due to the presence of acid-base nature oxygen functional groups on the material’s surface, such as carbonyle, carboxylate, and pyridine like structures which have been identified on carbon surfaces by Fourier transform infrared spectroscopy (FTIR) [8] and by liquid phase titration methods [9, 10]. There is evidence that the catalytic reactivity of metallic oxides mixed with condensed phases of organic precursors depends on the carbonaceous phases formed during the precursors pyrolysis, where the organic oxide provides the acid-basic properties that induce the carbon layer formation. Silica supported pyrolyzed PAN can show better textural properties, higher thermal stability and catalytic activity than unsupported PAN; then the former was proposed as a catalyst for the ODH of ethylbenzene in the vapor phase. This contribution is oriented to the preparation and characterization of PAN/SiO2 systems through different analytic techniques such as NH3-thermal programmed desorption (TPD), CO2-TPD, energy dispersive spectroscopy (EDS), and X-ray photoelectron spectroscopy (XPS) to elucidate the active sites of these materials.
Experimental
314 m2/g, 60/80 mesh silica gel for chromatography (SiO2 A) and 38 m2/g, 80/100 mesh silica Spherosil XOB (SiO2 B) were used as supports. Catalyst preparation was carried out by means of PAN dispersion over silica by acrylonitrile mass polymerization, using a monomer/support weight ratio equal to 3.6. Afterwards, the pyrolysis of the organic precursor (PAN) is performed, which is activated under nitrogen flow at 450°C before reaction. For the purpose of observing the effect on the physicochemical nature of the carbonaceous species, a side study of PAN pyrolysis with or without the presence of oxygen was made. The yield of pyrolyzed PAN per gram of catalyst in the PAN/SiO2 (A) system was 0.22 g with oxidative pretreatment, and 0.12 g without this treatment; while the PAN/SiO2 (B) material yields 0.30 g with the oxidative pretreatment and 0.18 g without this treatment. Thermal stability of the solids was determined in a Stanton Redcroft model STA-780 apparatus. PAN/SiO2 B-p showed a slightly higher thermal stability than PAN/SiO2 A-p when they were submitted to a programmed temperature analysis, from 30 to 700°C under an air flow; while the SiO2 B support also presented a higher (4%) thermal stability than SiO2 A [4]. The surface acidity and basicity of the solids were measured by means of TPD of ammonia and CO2, respectively, using an Altamira AMI-3 equipment. The IR analysis were carried out in a Nicolet spectrophotometer model 710, using the KBr wafer method. The C/N/O elemental analysis was carried out by EDS fitted to a Zeiss electronic microscope, model DSM 960A, and the surface chemical analysis by XPS in a Thermo VG Scientific spectrometer, model Escalab 250. The catalytic activity of the PAN/SiO2 materials was measured in a continuous flow fixed bed reactor system, on a temperature range between 340-400°C using 0.2 to 0.5 g of catalyst samples and EB flow rates of (15.8 to 38.7)x10-4 mol/h. The reaction products were analyzed by gas chromatography using a 10% Carbowax 20M/Chromosorb W packed column; ethylbenzene total conversion and styrene (ST) selectivity were calculated by the consumed EB moles and the feed EB moles ratio, and by the ST moles produced and the consumed EB moles ratio, respectively [11] .
Results and Discussion
Table 1 presents NH3-TPD and CO2-TPD data for PAN/SiO2 systems. Preoxidized solids (PAN/SiO2 A-p and PAN/SiO2 B-p) show a 50% higher total acidity compared to their not preoxidized counterparts, possibly due to the higher (15%) oxygen functional (phenolic and lactonic) groups concentration on the formers surface than on the not preoxidized materials. These functional groups were first determined by means of an acid-base titration method [4]. PAN/SiO2 A-p solid presents a NH3 adsorption two times higher than that of PAN/SiO2 B-p and unsupported PAN-p, principally due to the higher surface area of the former, since in a previous study SiO2 A and SiO2 B supports showed almost the same surface acidity as determined by NaOH titration method [12]. On the other hand, PAN/SiO2 B-p and PAN-p materials show a higher acid sites density, expressed in terms of μmol NH3/m2, in relation to the PAN/SiO2 A-p, and these last results showed a linear correlation when the catalytic activity of these PAN and PAN/SiO2 systems was expressed whether in terms of specific reaction rate (mol/h.g) or intrinsic reaction rate (mol/h.m2) in ethylbenzene ODH at 380-400°C [11]. With respect to basicity analysis, preoxidized PAN/SiO2 B-p sample presents a CO2 adsorption 1.3 times higher and more than two times higher than that of PAN/SiO2 A-p and PAN-p solids, respectively. In this case, the former also shows more than three times higher basic sites density and 1.3 times higher than the latter materials, and a linear correlation between that property and the catalytic activity in the ethylbenzene ODH was found, as discussed below. Table 1 also shows that the presence of pyrolyzed PAN on SiO2 A surface diminishes the acidity of this support, while an increase in basic sites concentration is observed when PAN is dispersed on the silica surface.
Table 1. Total acidity and basicity of pyrolyzed PAN/SiO2 materials determined by means of NH3-TPD and CO2-TPD, respectively.
Material
|
NH3, mmol/g
|
NH3, mmol/m2
|
CO2, mmol/g
|
CO2, mmol/m2
|
PAN/SiO2 A-p
|
30.07
|
0.25
|
76
|
0.7
|
PAN/SiO2 A
|
16.01
|
0.15
|
50
|
0.5
|
PAN/SiO2 B-p
|
15.51
|
0.39
|
100
|
2.5
|
PAN/SiO2 B
|
9.9
|
0.72
|
44
|
3.1
|
Figure 1 presents the IR spectra of pyridine adsorbed on unsupported pyrolyzed PAN, where the IR absorption band between 1460-1430 cm-1 of pyridine coordinated to Lewis acid sites was detected. This absorption band shows a characteristic doublette of pyrolyzed PAN, which can be related to two acid oxygen functional groups of this carbonaceous material, i.e. phenolic and lactonic type groups. That IR absorption band remains even though pyridine is evacuated at 200°C, this means that solid have weak and medium strength Lewis acid sites, according to Tanabe et al. [13]; on the other hand, the IR absorption signal corresponding to total acidity at 1490 cm-1 almost disappears at 200°C. Thermograms of preoxidized pyrolyzed PAN/SiO2 materials (not shown) present two NH3 desorption peaks, a small one at 298°C and another smaller one between 460-520°C, the latter can be the result of decomposition products of pyrolyzed PAN; whereas reference solid (PAN-p) shows two small NH3 desorption peaks at 298 and 350°C, respectively, which indicates this material also contains acid sites of moderate strength.
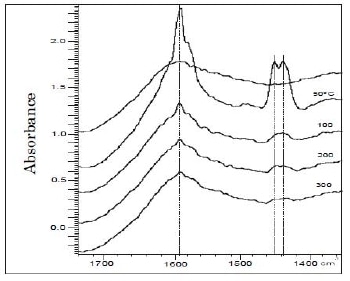
Figure 1. IR spectra of pyridine adsorbed on PAN-p.
Figure 2 presents CO2-TPD thermograms of preoxidized PAN/SiO2 systems. PAN/SiO2 A-p shows two CO desorption peaks, one relatively intense at 124°C and another one of low intensity at 465°C; while PAN/SiO2 B-p presents two CO2 desorption peaks at 121 and 485°C, respectively. On the other hand, unsupported PAN-p shows the first peak at 116 and the second one at 455°C; this means the PAN/SiO2 and PAN-p materials have a higher proportion of weak basic sites even though they also have an important quantity of strong basic sites, in accordance to Berteau et al. classification [14].
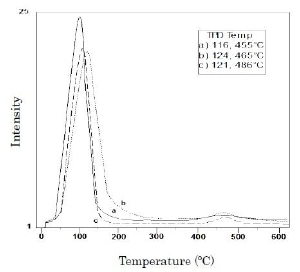
Figure 2. CO2 desorption thermograms: a) PAN-p, b) PAN/SiO2 A-p, c) PAN/SiO2 B-p.
The main peak between 116 and 124°C of these solids, corresponds to oxhydrile groups since the oxygen signal should appear after 200°C, in accordance to Tanabe et al. [13]. Pyridine chemisorption-IR on PAN/SiO2 catalysts did not register the presence of Bronsted acid sites, thus identified OH groups must have basic character, according to Tanaka et al. [15] and Di Cosimo et al. [16]; that is, these sites are capable to trap the ethyl group proton from the EB molecule. Table 2 shows elemental microanalysis (EDS) results of PAN/SiO2 systems. Preoxidized materials have a higher carbon concentration, PAN/SiO2 B-p compared to PAN/SiO2 A-p, and a higher oxygen concentration on PAN/SiO2 A-p in relation to PAN/SiO2 B-p. Nitrogen content is around 13 and 24 atomic percent; while unsupported PAN-p presents a higher carbon and nitrogen concentration, but it also has a lower oxygen concentration (O/C, N/C, and (O+N)/C atomic ratios were 0.38, 0.07 and 0.46, respectively) than supported solids [11].
Table 2. Elemental microanlysis (EDS) of PAN/SiO2 systems.
Material
|
PAN/SiO2 Ap
|
PAN/SiO2 A
|
PAN/SiO2 Bp
|
PAN/SiO2 B
|
Element/ atomic ratio
|
Atom, %
|
Atom, %
|
Atom, %
|
Atom, %
|
C
|
42.19
|
41.99
|
52.13
|
39.60
|
N
|
24.22
|
13.31
|
19.78
|
21.60
|
O
|
32.09
|
21.51
|
21.32
|
29.30
|
Si
|
1.48
|
23.25
|
6.76
|
9.4
|
N/C
|
0.57
|
0.32
|
0.38
|
0.54
|
O/C
|
0.76
|
0.51
|
0.41
|
0.74
|
(0+N)/C
|
1.33
|
0.83
|
0.79
|
1.28
|
preoxidized (p); not preoxidized ( )
The O/C, N/C and (O+N)/C atomic ratios are higher in PAN/SiO2 A-p respecting to its not preoxidized counterpart (PAN/SiO2 A), while this relationship is reversed for the PAN/SiO2 B-p. These results indicate the carbonaceous phase has a different C/N/O composition, depending on the support and pretreatment type used. The higher Si concentration on the PAN/SiO2 A surface compared to the PAN/SiO2 A-p one, could be due to the former which showed a carbonaceous layer poorer development during PAN pyrolysis without oxygen pretreatment, its particle size was around 0.5 μm while the particle size for the latter was ~ 1 μm, as demonstrated by means of SEM [4].
Figure 3 shows the XPS spectra for C1s deconvolution for PAN//SiO2 and PAN preoxidized systems. Supported materials spectra present an asimmetric peak around 285 eV markedly tailing on higher binding energy side, which suggests the presence of C-H and C-O bonds (288.5 eV) [17]. PAN/SiO2 A-p (a) main peak shows a prominent shoulder near 283 eV and another smaller one ∼ 288 eV; while PAN/SiO2 B-p (b) main peak presents a small shoulder close to 283 eV, and at least another one around 287 eV. On the other hand, unsupported PAN-p (c) shows the main peak at 283.3 eV and at least a shoulder on the high binding energy side, near 285.3 eV which corresponds to PAN/SiO2 B-p main peak. Binding energy (eV) Figure 3. XPS spectra for C1s deconvolution for: a) PAN/SiO2 A-p, b) PAN/SiO2 B-p, c) PAN-p. Takahagi and Ishitani [18] studied the surface functional groups on carbon and graphite fibers by means of XPS. From C1s spectra three compounds with different chemical displacement were identified: oxhydrile -OH, carbonyle –C=O and carboxyl –COOH groups at 286, 287 and 288.6 eV, respectively. The peak at 283.2 eV corresponds to graphite carbon, according to Laszlo et al. [19], who carried out a study on synthetic carbon from pyrolyzed PAN. Burg et al. [20] in urea modified activated carbons, ascribe the peak at 286.3 eV (Figure 3) to carbon linked to oxygen by simple bond: C-OH, C-O-C or C-OH in phenol; the peak at 285.3 eV is related to α position carbon in –COOR, and β or γ position in pyridinic nitrogen (N6). This peak is assigned to carbon in phenolic, ether and C=N groups, in accordance to Laszlo et al. [19] and Nakajima and Koh [21]. A peak at 287.4 eV was attributed to carbon joined to oxygen by double bond: -C=O, -C=O(OH), Ar-CO-R, which is in agreement with Takahagi and Ishitani [18] and Laszlo et al. [19] assignments (carbon in carbonyle or quinone groups), who ascribe also the peak at 288.7 eV to carbon in carboxylic or ester C=O-OR, lactones and carboxylic anhydride groups.
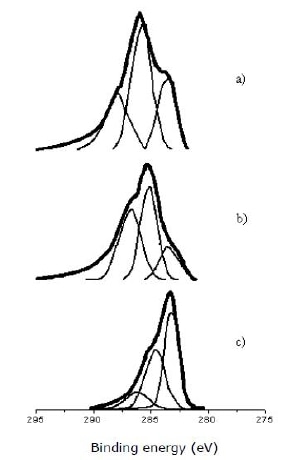
Figure 3. XPS spectra for C1s deconvolution for: a) PAN/SiO2 A-p, b) PAN/SiO2 B-p, c) PAN-p..
Considering the results mentioned in Robles et al. [11], PAN/SiO2 A-p carbonaceous phase contains: oxhydrile (50%) and carboxylic or ester groups (24%), as well as graphitic carbon (26%); while PAN/SiO2 B-p contains carbonyle/quinone (41%), ester and pyridine like groups (41%), and graphitic carbon (18%). On the other hand, reference solid (unsupported PAN-p) contains carbonyle/quinone (12%), ester and pyridinic groups (39%), and graphitic carbon (49%). Figure 4 shows the effect of PAN/SiO2 C/N/O (EDS) concentration on the basic sites density (Db, µmol CO2/m2) and on the reaction intrinsic rate (Ri, mol EB/h-m2) in ODH of EB at 400°C and W/F 177 g cat/mol/h. The catalysts abscise axis order is: PAN-p < PAN/SiO2 B-p < PAN/SiO2 A < PAN/SiO2 B < PAN/SiO2 A-p. Db and Ri show a similar behavior when (O+N)/C atomic ratio increases, presenting two maxima values in these parameters that correspond to PAN/SiO2 B-p and PAN/SiO2 B materials, respectively. Most active catalysts have a (O+N)/C concentration between 0.5 and 0.8. Considering preoxidized solids only, the Db and Ri maximum still corresponds to PAN/SiO2 B-p sample, probably due to its higher oxygen functional groups concentration [4], more precisely to its higher carbonyle/quinone and pyridine like structures concentration compared to PAN-p and PAN/SiO2 A-p.
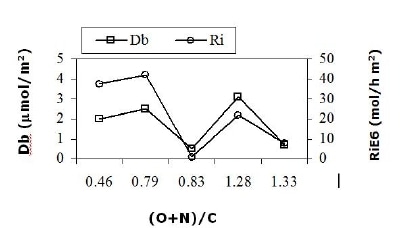
Figure 4. (O+N)/C (EDS) composition influence PAN/SiO2 materials on basic sites density and the intrinsic reaction rate in ethylbenzene ODH at 400°C and 177 g cat/mol/h.
Figure 5 presents the surface oxygen composition (O1s/O1b) effect on the basic sites density Db and the intrinsic reaction rate Ri for PAN base preoxidized materials, whose order on the abscise axis is: PAN/SiO2 A-p < PAN/SiO2 B-p < PAN-p. O1s/O1b is the atomic ratio between XPS and EDS oxygen concentration, respectively. There is a similar behavior in Db and Ri when oxygen surface composition increases from 0.8 to 2.0. This fact suggests that there is an O1s/O1b atomic ratio optimum range between 1.5 and 1.7, corresponding to the most active (PAN/SiO2 B-p) catalyst.
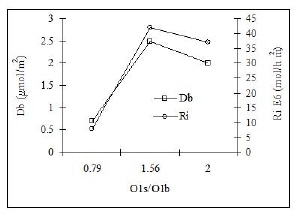
Figure 5. Effect of PAN/SiO2 oxygen surface composition on basic sites density and the intrinsic reaction rate in ethylbenzene ODH at 400°C and 177 g cat/mol/h.
Figure 6 shows the effect of PAN/SiO2 basic sites density on the intrinsic reaction rate in ODH of EB at 400°C. It is observed a linear correlation between both parameters to a basic sites concentration of 2.5 µmol CO2/m2 corresponding to PAN/SiO2 B-p, which confirms that basic nature functional groups (carbonyle, carboxylate and pyridine like groups) can be the active sites for the EB oxidative dehydrogenation. The atypical point which corresponds to not preoxidized PAN/SiO2 B solid, even if this one has the highest basic sites density (Db = 3.1 µmol CO2/m2), shows an EB molecules transformation rate (TOF) 2.2 times lower than PAN/SiO2 B-p.
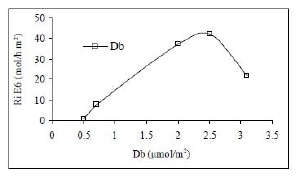
Figure 6. Effect of PAN/SiO2 basic sites density on the intrinsic reaction rate in ethylbenzene ODH at 400°C and 177 g cat/mol/h.
XPS analysis showed the basic functional groups on pyrolyzed PAN/SiO2 systems which are: carbonyl/quinone, carboxylic or esters and pyridine like structures, also detecting graphite carbon. Catalytic activity measurements suggest PAN/SiO2 active sites for ODH of EB are the basic nature functional groups such as carbonyl/quinone, carboxylate, pyridinic, and graphitic carbon, ether and carboxylic anhydride. It is well known that moderate basic strength OH groups are proton trap centers [11], while carboxylate ions are moderately basic and tends to combine with protons [22], and pyridinic groups which are hydrogen acceptor centers [23]. However, oxhydrile ions and Lewis weak acid sites determined by means of NH3-TPD and pyridine chemisorption, as well as phenolic and lactonic groups detected by Boehm titration method, respectively, can play an important role in the ethylbenzene reaction mechanism in accordance to Solsona et al [24], who studied VPO catalysts in ODH of propane. These authors consider that basic sites carry out their dehydrogenating function and help the products to rapidly desorb. The former authors and Katz [25] explain the way acid and basic sites act when they are close to each other (acid-base pair) on catalysts surface, in basic sites demanding reactions like Aldol and Knoevenagel condensations. Acid sites activate EB molecule which commonly is the limiting reaction step, and basic sites trap the ethyl group protons. Generally, basic sites are lattice oxygen in case of transition oxide metals, and oxygen of functional groups in carbonaceous solids.
Conclusions
NH3-TPD results confirmed the oxygen surface groups existence of weak and moderate acid strength and CO2-TPD analysis registered the presence of moderate basic strength OH ions. Pyridine adsorption IR results indicated only the presence of Lewis type weak acid sites on the PAN/SiO2 systems surface. XPS analysis revealed the most active pyrolyzed PAN/SiO2 catalyst contains: 40% carbonyle/quinone, 40% esters and pyridinic groups, and 20% graphitic carbon; while unsupported PAN-p contains 12% carbonyle/quinone, 39% esters and pyridine like structures, and 49% graphitic carbon. Catalytic activity measurements indicate PAN/SiO2 materials active sites for oxidative dehydrogenation of ethylbenzene are the basic nature oxygen surface groups such as carbonyle/quinone, carboxylate, esters, as well as pyridine like structures, which act in a concerted way with acidic sites (phenolic and/or lactonic), where the latters activate the EB molecule and the formers carry out the dehydrogenating function and facilitate the styrene desorption.
References
- 1. P. N. Degannes and D. M. Ruthven, “The Oxidative Dehydrogenation of Ethylbenzene to Styrene”, The Can. J. Chem. Eng., 57 (1979) 627-630.
- 2. Mc Dougal G. F., “The Physical Nature and Manufacture of Activated Carbon”, J. South Afr. Inst. Min. Metall., 91, [4] (1991) 109-120.
- 3. A. E. Lisovskii and Ch. Aharoni, “Carbonaceous Deposits as Catalysts for Oxydehydrogenation of Alkylbenzenes”, Catal. Rev. Sci. Eng., 36, [1] (1994) 25-74.
- 4. A. S. Robles, A. J. Escobar, M. A. Castillo, B. J. Navarrete and R. G. Sandoval, “Preparación y Caracterización de Catalizadores Orgánicos Soportados en Sílice”, Actas XV Simp. Iberoam. Catal., (1996) 373-378.
- 5. Y. Iwasawa, H. Nobe and S. Ogasawara, “Reaction Mechanism for Styrene Synthesis Over Polynaphtoquinone”, J. Catal., 31 (1973) 444-449.
- 6. G. C. Grunewald and R. S. Drago, “Oxidative Dehydrogenation of Ethylbenzene to Styrene Over Carbon-based Catalysts”, J. Mol. Catal., 58 (1990) 227-233.
- 7. H. P. Boehm, “Some Aspects of Surface Chemistry of Carbon Black and Other Carbons”, Carbon, 32, [5] (1994) 759-769.
- 8. G. C. Grunewlad, R. S. Drago and J. L. Clark, “Preparation, Characterization, and Reactivity of a Novel Heterogeneous Catalyst for the Reduction of CO”, J. Mol. Catal., 60 (1990) 239-253.
- 9. H. P. Boehm and M. Voli, Carbon, 8, 227 (1970)
- 10. A. S. Robles, R. G. Sandoval, S. M. E. Llanos and E. J. M. Domínguez, “Physicochemical Charac-terization and Catalytic Activity of SiO2 Supported Organic Catalysts in the Oxidative Dehydroge-nation of Ethylbenzene”, 15th Meeting North Am. Catal. Society, (1997), pp. 171.
- 11. A. S. Robles, “Obtención de Estireno Por Deshidrogenación Oxidativa del Etilbenceno con un Catalizador de Poliacrilonitrilo Pirolizado/SiO2”, Tesis de Doctorado, División de Estudios de Posgrado e Investigación, Instituto Tecnológico de Cd. Madero, (2005) 37-41.
- 12. A. J. Escobar, “Preparación y Caracterización de Catalizadores Poliméricos Pirolizados”, Tesis de Maestría, División de Estudios de Posgrado del Instituto Tecnológico de Cd. Madero, (1993).
- 13. K. Tanabe, M. Misono, Y. Ono and H. Hattori, “New Solids and Bases, their Catalytic Properties” Kodansha LTD, Tokio and Elsevier Sci. Publ., Amsterdam, (1989) 5-13.
- 14. P. Berteau, M. A. Kellens and B. Delmon, “Acid-base Properties of Modified Aluminas”, J. Chem. Soc. Faraday Trans., 87, [9] (1991) 1425-1431.
- 15. T. Tanaka, H. Kumagai, H. Hattori, M. Kudo and S. Hasegawa, “Generation of Basic Sites on TiO2 by Reduction with H2”, J. Catal., 127 (1991) 221-226.
- 16. J. L. Di Cosimo, V. K. Díez, M. Xu, E. Iglesia and C. R. Apesteguía, “Structure and Surface and Catalytic Properties of Mg-Al Basic Oxides”, J. Catal., 178 (1998) 499-510.
- 17. R. Ch. Bansal, J. B. Donet and F. Stoeckli, “Active Carbon”, Marcel Dekker, Inc., New York and Basel, (1998) 92-105.
- 18. T. Takahagi and A. Ishitani, “XPS Studies by Use of the Digital Difference Spectrum Technique of Functional Groups on the Surface of Carbon Fiber”, Carbon, 22, [1] (1984) 43-46.
- 19. K. Laszlo, K. Josepovits and E. Tombacz, “Analytical Sciences”, (2001) 17, Supl., 1741-1744.
- 20. P. Burg, P. Fydrych, D. Cagniant, G. Nanse and J. Bimer, “The Characterization of Nitrogen-enriched Activated Carbons”, Carbon, 40 (2002) 1521-1531.
- 21. T. Nakajima and M. Koh, “Synthesis of High Crystalline Carbon-nitrogen Layered Compound by CVD using Nickel and Cobalt Catalysts”, Carbon, 35, [2] (1997) 203-208.
- 22. R. T. Morrison and R. N. Boyd, “Organic Chemistry”, 5th Edition, Allyn and Bacon, Inc. Newton, Mass, USA, (1987).
- 23. A. Schraut, G. Emig and H. G. Sockel, “Composition and Structure of Active Coke in the Oxydehy- Drogenation of Ethylbenzene”, Applied Catalysis, 29 (1987) 311-326.
- 24. B. Solsona, V. A. Zazhigalov, J. M. López Nieto, I. V. Bacherikova and E. A. Diyuk, “Oxidative de-hydrogenation of Ethane on Promoted VPO Catalysts”, Appl. Catal. A: General 249, (2003) 81-92.
- 25. A. Katz, 5th Internat. Simp. on Acid Base Catalysis, Puerto Vallarta, México, (2005).
Contact Details
Ramos-Galván C., Sandoval-Robles G., Robles-Andrade S., García-Alamilla R.
Instituto Tecnológico de Ciudad Madero, División de Estudios de Posgrado e Investigación
J. Rosas esquina con J. Urueta s/n, Col. Los Mangos, 89440 Ciudad Madero, Tam., México
E-mail: [email protected]
Llanos-Serrano M. E., Navarrete-Bolaños J. and Domínguez-Esquivel J. M.
Instituto Mexicano del Petróleo, Ingeniería Molecular, Eje Central L. Cárdenas 152, 07730 México, D. F.
This paper was also published in print form in “Advances in Technology of Materials and Materials Processing”, 10[1] (2009) 39-46.