Nonlinear optical pump-probe microscopy is a new field for 3D high resolution imaging in modern microscopy. The imaging contrast relies on the characteristic spectroscopic properties of specific molecules, i.e. their specific internal energy level structure.
Nonlinear light-matter interactions are used to selective probe this spectroscopic fingerprint, yielding an image that reveals the spatial distribution of the targeted molecule. Depending on the method, the stimulated energy transitions can be molecular electronic transitions in the visible range, or vibrational resonances of molecular bonds in the infrared range.
A typical image with 512 by 512 pixels covers an area of 100 by 100 µm. Images are consecutively recorded at distinct focal planes with a typical vertical spacing of 1 µm to obtain high resolution 3D images.
Compared to other nonlinear optical imaging methods like fluorescence and incoherent Raman microscopy, stimulation and readout of the nonlinear interactions is achieved by at least two pulses of well defined properties: a pump and a probe pulse. The suppression of incoherent processes such as spontaneous emission is one of the key advantages of this stimulated readout.
High excitation laser power in combination with sensitive detection results in an efficient overall yield of the nonlinear interaction, enabling fast image acquisition times. Photo damage on the sample, which is caused by the high powered laser is reduced due to the short laser exposure time. Compared to fluorescence imaging, particular marker molecules are not required for sample preperation.
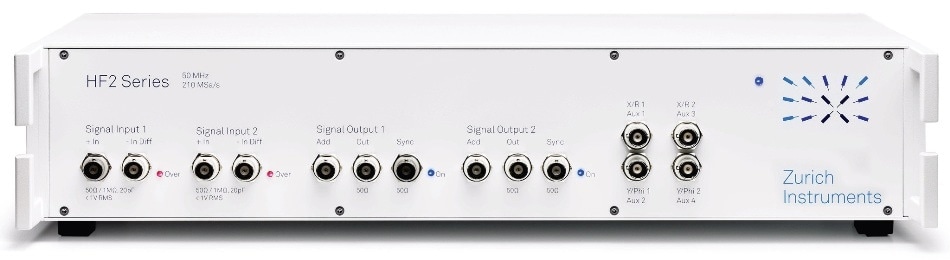
Reducing the image acquisition time, which is often directly related to detection sensitivity, is one of the key goals of the current research. Modern scanning microscope technology enables acquisition times at up to 30 fps for a 512 by 512 pixel image [1] and such acquisition times facilitate rapid 3D imaging. However, it is possible to record 2D images at video-rates to analyze dynamics in complex biosystems on a microscopic scale.
Nonlinear optical pump-probe microscopy has the ability to perform 3D imaging within tissues, making it a potential technique for non-invasive imaging in biomedicine to provide complementary data to currently applied fluorescence and magnetic resonance imaging techniques.
Table 1. Example wavelengths as used in [2] for C-D and C-H bond vibration
Vibration |
C-H bond |
C-D bond |
Ω |
2875 cm-1 |
2100 cm-1 |
λP |
830 nm |
830 nm |
λS |
1090 nm |
1005 nm |
λA |
670 nm |
707 nm |
Coherent Raman Microscopy
The vibrational fingerprint for molecule-selective imaging is utilized in coherent Raman microscopy. Coherent anti-Stokes Raman scattering (CARS) and stimulated Raman scattering (SRS) are discussed in this article.
Figure 1 depicts an illustration of the corresponding multi-photon process for SRS and CARS. Both techniques involve coherent excitation of molecule vibrations by simultaneously applying near-infrared Stokes and visible pump pulses with a resonant frequency difference Ω. The Stokes and pump pulses need to be synchronized in time and must obey a well-established phase relationship to trigger these multi-photon processes.
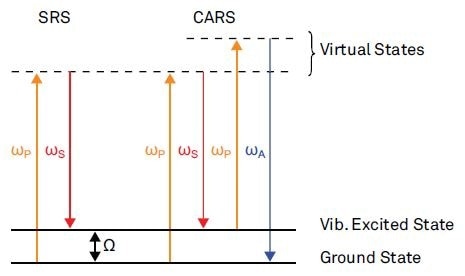
Figure 1. Nonlinear optical pump-probe microscopy is a new field for 3D high resolution imaging in modern microscopy.
SRS probes the vibrational excited states by determining the energy exchange between the molecule and the laser field caused by the nonlinear interaction. This energy exchanges manifests in an intensity gain in the Stokes pulse (stimulated Raman gain) and an intensity loss in the probe pulse (stimulated Raman loss).
The nonlinear interaction is continuously turned on and off by intensity modulation of the Stokes pulses for measurements. The stimulated Raman loss on the pump pulses appears modulated and can be determined with a lock-in amplifier. This detection method, also called modulation transfer, enables fast imaging. An example is the video-rate in-vivo SRS imaging demonstrated by Saar et al [3] .
Another possible implementation of modulation transfer in SRS microscopy is to modulate the pump pulse and detect the stimulated Raman gain on the Stokes pulse.
CARS probes the excited vibrational resonances by monitoring the stimulated anti-Stokes emission. However, the sensitivity of direct detection of the anti-Stokes emission is limited by a weak background contribution to the anti-Stokes emission that is not related to any vibrational resonances. The review by Day et al [4] mentions two different lock-in schemes for background free CARS imaging with high-sensitivity.
The first lock-in technique is frequency modulated CARS (FM-CARS) and it was implemented for the first time by Ganikanov et al [5]. Frequency modulation of the pump pulses modulates the vibrational resonance frequency Ω. Then, demodulated anti-Stokes signal represents the frequency derivative of the anti-Stokes emission at the excitation frequency. Hence, the background contribution is rejected because of its flat frequency response.
The second lock-in technique is interferometric CARS, as implemented by Potma et al [6] for the first time. There is interference between phase-modulated local oscillator pulses at the anti-Stokes frequency and the anti-Stokes emission of the imaging specimen.
As there is a quadrature phase relation between the resonant anti-Stokes contribution and the background, the correct adjustment of the local oscillators optical phase leads to a background-free vibrational absorption signal. The phase of the vibrational response is fully resolved for chemical selectivity amongst overlapping resonances from various molecular bonds, according to the recent experiments by Jurna et al [7].
Nonlinear Absorption Microscopy
The imaging contrast in nonlinear absorption microscopy is based on characteristic molecular electronic transitions. In the modulation transfer technique, the nonlinear interaction is detected by the energy exchange between the laser field and the molecule. A recent review of Min et al [8] provides a number of nonlinear absorption examples for imaging. Figure 2 shows the absorption process for two of these.
Figure 2a shows the absorption of two photons by passing a virtual state, provided that the sum of their frequency matches an excited state. This process is called two photon absorption (TPA).
The excitation pulses must be synchronized in time and obey a well-established phase relationship. It is also possible to detect TPA when the wavelength of the two photons is the same, provided that both interacting photons are intensity modulated. As explained by Ye et al [9], the nonlinear absorption process leads to a response at twice the modulation frequency.
Figure 2b displays a potential process occurring in transient absorption (TA) imaging. Both laser frequencies are tuned to an electronic transition and a femtosecond time delay is introduced between the pump and the probe pulses.
Then, the transient absorption is the absorption of the probe pulse due to the preceding pump pulse. Ground-state electrons are excited by the pump pulse for the configuration depicted in Figure 2b.
When the electrons are in the excited state, the probe pulse can see a ground-state population smaller than that without the pump pulse. As a result, the absorption of the probe pulse is less in the presence of the pump pulse compared to that in the absence of the pump pulse.
It is also possible to interrogate the decay dynamics of the excited state by altering the delay between the pulses, as applied in pump-probe spectroscopy to probe femtosecond electron dynamics.
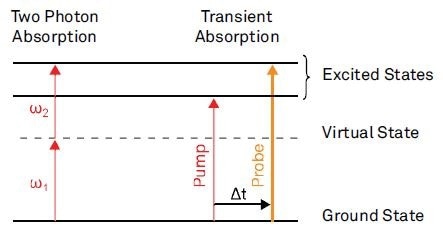
Figure 2. Examples for nonlinear absorption processes.
Example Experiments
Stimulated Raman Scattering Microscopy
Delong Zhang and Mikhail Slipchenko from the group of Ji-Xin Chengs at Purdue University built an SRS imaging setup, as described in [2]. Figure 3 shows the parts relevant to modulation transfer SRS experiments and their interconnection.
An optical parametric oscillator (OPO) pumped by a femtosecond Ti:Sapphire laser oscillator provides the two tunable excitation pulses (pump and Stokes). Subsequently, an acousto-optic modulator (AOM) is used to intensity modulate the Stokes pulses at 5.4 MHz. The resulting Stokes pulses are then spatially and temporally overlapped with the pump pulses. The lock-in amplifier provides the 5.4 MHz reference carrier.
![SRS microscope as used by Zhang et al [2] with parts related to backward fluorescence imaging omitted. The list on the left hand side mentions all the involved optical frequencies. Wavelength Generation: pump pulses generated with a femtosecond pump laser (repetition rate 80 MHz). Tunable pump (680 - 080 nm) and Stokes (1000 - 1600 nm) are provided by an optical parametric oscilla tor (OPO). Synchronization, Modulation: time synchronization of the pulse trains with a delay stage and intensity modulation at 5.4 MHz with an acousto optic modulator (AOM). Modulation carrier from HF2LI fed to driver. Laser Scan Microscope: microscope with laser steering controlled by the Microscope controller.](https://www.azom.com/images/Article_Images/ImageForArticle_13263_45008376119513892450.jpg)
Figure 3. SRS microscope as used by Zhang et al [2] with parts related to backward fluorescence imaging omitted. The list on the left hand side mentions all the involved optical frequencies. Wavelength Generation: pump pulses generated with a femtosecond pump laser (repetition rate 80 MHz). Tunable pump (680 - 080 nm) and Stokes (1000 - 1600 nm) are provided by an optical parametric oscilla tor (OPO). Synchronization, Modulation: time synchronization of the pulse trains with a delay stage and intensity modulation at 5.4 MHz with an acousto optic modulator (AOM). Modulation carrier from HF2LI fed to driver. Laser Scan Microscope: microscope with laser steering controlled by the Microscope controller.
A high numerical aperture objective is used to focus the excitation beams into the sample and another microscope objective is used to collect both the SRS and CARS signals generated from the sample, in the forward direction.
After optically filtering the pump pulses from the output pulses, they are measured by a photodiode and demodulated by the HF2LI to measure the stimulated Raman loss. The demodulated signal is transmitted to the microscope controller via the HF2LI’s auxiliary output.
One innovative feature of the microscope presented in [2] is the application of femtosecond laser pulses instead of the widely used picosecond pulses. The femtosecond laser pulses are capable of exciting isolated vibrational resonances better, due to the increased excitation bandwidth of shorter pulses.
Photodamage on biological samples is an important issue because of the higher peak power of the femtosecond laser pulses. The authors addressed this issue by balancing the excitation power to the near-infrared Stokes pulses (40 mW), where possible photodamage is relatively smaller compared to that for the visible pump pulses (10 mW). Also, images are collected at high-speed with high-sensitivity. The pixel dwell time, which is the exposure time to the laser of one sample pixel, remains below 4 µs.
The synthesis of lipid droplets (LD) consisting of fatty acids (FA) for energy storage within cells, as previously studied by Slipchenko et al [10] using Raman microscopy is one of the areas of focus of their study. LDs synthesized from endogenous glucose present within the cell are determined by imaging C-H bonds at a Raman shift of 2875 cm-1.
Deuterated exogenous FA is added with a characteristic response from C-D bonds at a Raman shift of 2100 cm-1 to enable SRS imaging to differentiate between endogenous and exogenous FA within LD. This principle is shown in Figure 4.
![Adapted with permission from [2]. Copyright 2011 American Chemical Society. The figure shows SRS and transmission images of CHO cells incubated for 7 hours in the cell culture medium supplemented with 100µM deuterated palmitic acid (exogenous FA with C-D vibration). Figure 4C shows an SRS image of the C-D vibration and indicates the presence of exogenous FA in LD and cell membranes. Figure 4G shows an SRS image at the C-H vibration of the same cells and shows the distribution of endogenous FA in cells. Notably, the location of LD in 4C and 4G indicates that even when cells are supplemented with exogenous FA, intra-cellular LD contain both endogenous and exogenous FA. This suggests that the cell still synthesizes endogenous FA to facilitate the LD formation. The transmission image in Figure 4K lacks any chemical information found in the SRS images. Scale bar: 5 µm.](https://www.azom.com/images/Article_Images/ImageForArticle_13263_45008376126134269132.jpg)
Figure 4. Adapted with permission from [2]. Copyright 2011 American Chemical Society. The figure shows SRS and transmission images of CHO cells incubated for 7 hours in the cell culture medium supplemented with 100µM deuterated palmitic acid (exogenous FA with C-D vibration). Figure 4C shows an SRS image of the C-D vibration and indicates the presence of exogenous FA in LD and cell membranes. Figure 4G shows an SRS image at the C-H vibration of the same cells and shows the distribution of endogenous FA in cells. Notably, the location of LD in 4C and 4G indicates that even when cells are supplemented with exogenous FA, intra-cellular LD contain both endogenous and exogenous FA. This suggests that the cell still synthesizes endogenous FA to facilitate the LD formation. The transmission image in Figure 4K lacks any chemical information found in the SRS images. Scale bar: 5 µm.
In this study, 3D SRS images of an anesthetized C. elegans worm were also collected at the C-H vibration. From these images, a 3D map of the LD distribution within the worm is created. Overall, 50 frames are acquired at different elevations with 512 by 512 pixels with a pixel dwell time of 2 µs.
Considering the repositioning of the beams within each frame, it takes only 55 seconds to acquire the 150 x 150 x 50 µm image. Also, the SRS microscope can be used with other imaging modalities like two photon fluorescence, which can be detected in the backward direction without using the lock-in amplifier.
Transient Absorption Microscopy
Desiré Whitmore and Yong Wan in the Potma Group at UC Irvine constructed a nonlinear microscope capable of performing transient absorption (TA) imaging with an HF2LI for signal acquisition [11]. Table 2 lists the related femtosecond pulses with a repetition rate of 80 MHz.
Table 2. Components of transient absorption process
Excitation pulses |
Output pulses |
ω1: pump pulse (mod) |
ω1: pump pulse (mod) |
ω2: probe pulse |
ω2: probe pulse |
|
ω2: transient absorption (mod) |
The wavelength of the probe pulse and pump pulse is 943 nm and 820 nm, respectively. Pump pulses pass the AOM and the probe pulses pass the delay stage to adjust the interpulse delay, as shown in Figure 3. The excitation powers are 1 mW for the pump pulse and 0.5 mW for the probe pulse. The modulation frequency is 1.5MHz.
Once interaction with the sample occurs, the transient absorption on the probe pulse is isolated from the output pulses by optical filtering and demodulation by the HF2LI. The signal is then transmitted to the microscope controller by the auxiliary outputs of the HF2LI.
The wavelength configuration utilized for TA by Desiré Whitmore uses stimulated emission in metallic structures as imaging contrast. As a result, individual single walled carbon nanotubes (SWNT) with metallic properties can be visualized.
Although carbon nanotubes have the potential to offer innovational solutions in many fields of technology, they are difficult to synthesize with specific material properties. They must be characterized individually to select SWNTs with specific material properties at the end of the fabrication process.
Nonlinear optical pump-probe microscopy with the ability to study fast electron dynamics by TA can be used for this purpose. The optical response is weak due to the very small size of SWNTs, requiring long image acquisition times. As the maximum pixel dwell time is limited by photodamage, frame based averaging is applied rather than a single scan with long acquisition time. Figure 5 illustrates SWNT imaging with a pixel dwell time of 20 µs.
![Adapted with permission from [11]. Copyright 2011 Desiré Whitmore. Figure 5a is a scanning electron microscope (SEM) image of synthesized SWNT with Titanium pads for electrical characterization. While the SEM resolves individual SWNT, no information concerning the electronic properties is obtained. Figure 5b shows a TA image of the same region. As the response from SWNT depends on the optical polarization of the laser pulses, only horizontally aligned SWNT are visible. By changing the interpulse delay, the electron dynamics of individual metallic SWNT are studied. The pads are resolved in the TA image due to their metallic properties. The pixel dwell time was 20 µs and a longer pixel dwell time of 100 µs resulted in photodamage of the SWNT. Figure 5c shows a transmission image of the same region and does not show any signature from SWNT.](https://www.azom.com/images/Article_Images/ImageForArticle_13263_450083761329976846153.jpg)
Figure 5. Adapted with permission from [11]. Copyright 2011 Desiré Whitmore. Figure 5a is a scanning electron microscope (SEM) image of synthesized SWNT with Titanium pads for electrical characterization. While the SEM resolves individual SWNT, no information concerning the electronic properties is obtained. Figure 5b shows a TA image of the same region. As the response from SWNT depends on the optical polarization of the laser pulses, only horizontally aligned SWNT are visible. By changing the interpulse delay, the electron dynamics of individual metallic SWNT are studied. The pads are resolved in the TA image due to their metallic properties. The pixel dwell time was 20 µs and a longer pixel dwell time of 100 µs resulted in photodamage of the SWNT. Figure 5c shows a transmission image of the same region and does not show any signature from SWNT.
User Benefits
Compared to other commercially available devices, the Zurich Instruments HF2LI lock-in amplifier has features that are essential for high-speed nonlinear optical pump-probe microscopy:
- Fastest filter time constant of 780 ns
- 120 dB dynamic reserve for best-in-class signal-to-noise ratio
- Frequency range up to 50 MHz
- Auxiliary outputs sampled at 1 MS/s
- Up to 6 demodulators in one lock-in unit
- Constructed with the most advanced integrated components available in the market
Modulation frequencies are always below 40 MHz with a typical pulsed laser repetition rate of 80 MHz. The frequency range of the HF2LI is a perfect match for these conditions. It is possible to set the demodulation bandwidth as high as 200 kHz for fast scanning speeds, by selecting a first order filter with a time constant of 780 ns.
For fast output and acquisition of the demodulated signals, unnecessary distortions can be avoided by updating the auxiliary outputs of the HF2LI every microsecond with time equidistant samples. A 200 kHz filter suppresses aliasing on these outputs.
At the auxiliary outputs of HF2LI, the maximum effective demodulation bandwidth is 147 kHz, taking into account the superposition of the two filter transfer functions. Alternatively, using USB cable, the 200 kHz bandwidth can be achieved with digital sample transfer to the computer.
The achievable imaging frame rate with an HF2LI employed for signal acquisition for different resolutions is summarized in Table 3. The number of pixels per frame is shown by the left-most column. The 5% Rate is, for example the frame rate, where the demodulation filter causes a 5% increase in the smallest resolvable feature size.
It is assumed for all of these frame rates that the smallest resolvable feature is sampled by two pixels. For instance, 128 x 128 µm is the size of the 512 x 512 pixel frame. A zero time is assumed for the frame rates listed in Table 3 for subsequent frames acquisition. The detailed calculations can be found in [12].
Table 3. Achievable imaging frame rates with HF2LI
Frame pixels |
5% Rate |
10% Rate |
20% Rate |
512 x 512 |
1.2 fps |
1.7 fps |
2.3 fps |
256 x 256 |
4.9 fps |
6.7 fps |
9.4 fps |
175 x 175 |
10 fps |
14 fps |
20 fps |
It is possible to achieve high-speed microscopy with 10 or more frames per second with a minimal trade-off of special resolution. In addition to coherent Raman and nonlinear absorption microscopy, nonlinear pump-probe imaging techniques like photothermal microscopy can also benefit from the fast detection performance.
The HF2LI also has two signal inputs and six demodulators, which can be employed for various detection methods. One example is simultaneously measuring modulation transfer, which is determined on the first channel, and single beam two photon absorption, which is detected on the second channel of the HF2LI at any arbitrary harmonic of the modulation frequency.
Accordingly, it is necessary to extend the optical setup to detect the collected pump-probe pulses simultaneously. Pseudoheterodyne detection method [13] is another example, where the vibrational phase in the interferometric CARS setup is measured with optical phase modulators.
Acknowledgements
Zurich Instruments thanks Mikhail Slipchenko from the Purdue University and Desiré Whitmore from UC Irvine (currently UC Berkeley) for their support and sharing results from their measurement setups, which are shown in the example experiments of this application note.
References and Further Reading
- Evans, Potma, Puoris’haag, Cote, Lin, Xie, Chemical imaging of tissue in vivo with video-rate coherent anti- Stokes Raman scattering microscopy, PNAS, 102 (46) 16807-16812, 2005
- Zhang, Slipchenko, Cheng, Highly sensitive vibrational imaging by femtosecond pulse stimulated Raman loss, J. Phys. Chem. Lett., Vol 2, 1248 - 1253, 2011
- Saar, Freudiger, Reichman, Stanley, Holtom, Xie, Video-rate molecular imaging in vivo with stimulated Raman scattering, Science, Vol 330, 2010
- Day, Domke, Rago, Kano, Hamaguchi, Vartiainen, Bonn, Quantitative coherent anti-Stokes Raman scattering (CARS) microscopy, J. Phys. Chem. B, Vol 115, 2011
- Ganikhanov, Evans, Saar, Xie, High-sensitivity vibrational imaging with frequency modulation coherent anti- Stokes Raman scattering (FM CARS) microscopy, Optics Letters, Vol 31 No 12, 2006
- Potma, Evans, Xie, Heterodyne coherent anti-Stokes Raman scattering (CARS) imaging, Optics Letters, Vol 31 No 2, 2006
- Jurna, Garbacik, Korterik, Herek, Otto, Offerhaus, Visualizing resonances in the complex plane with vibrational phase contrast coherent anti-Stokes Raman scattering, Anal. Chem, Vol 82, 2010
- Min, Freudiger, Lu, Xie, Coherent nonlinear optical imaging: Beyond fluorescence microscopy, Annu. Rev. Phys. Chem., Vol 62, 507-530, 2011
- Ye, Fu, Warren, Nonlinear absorption microscopy, Photochemistry and Photobiology, Vol 85, 631 - 645, 2009
- Slipchenko, Le, Chen, Cheng, High-speed vibrational imaging and spectral analysis of lipid bodies by compound raman microscopy, J. Phys. Chem. B, Vol 113, 7681 - 7686, 2009
- Whitmore, Design and construction of linear and nonlinear optical systems for measurements of nanomaterials: Towards chemistry at the space-time limit, Ph.D. Thesis, University of California, Irvine, 2011
- Derivations shown in Zurich Instruments Blogs, www.zhinst.com
- Zurich Instruments application note on pseudoheterodyne detection in optics, www.zhinst.com
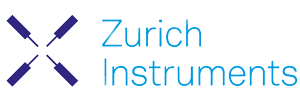
This information has been sourced, reviewed and adapted from materials provided by Zurich Instruments.
For more information on this source, please visit Zurich Instruments.