To address the worldwide energy crisis and environmental degradation caused by fossil fuels, it is critical to create ecologically friendly, safe, and dependable energy storage devices. This has been discussed in the journal Electrochimica Acta.
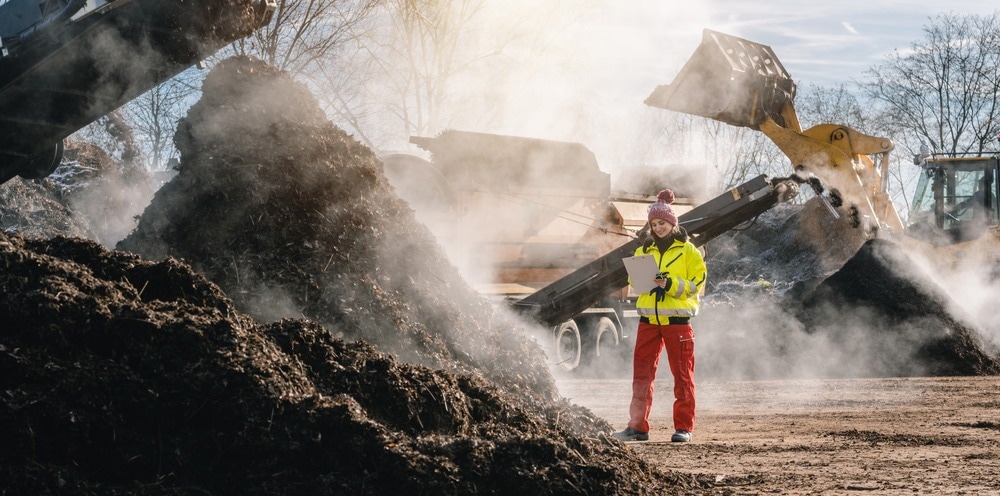
Study: Green Bridge between Waste and Energy_Conversion the Rotten Wood into Cathode for Functional Zn-Air Battery. Image Credit: Kzenon/Shutterstock.com
Due to their high specific energy, consistent discharge performance, and different presentation modes, carbon materials can be employed as cathode materials for Zn-air batteries (ZAB), but their low intrinsic activity limits their electrochemical applicability.
Carbon materials offer a wide range of applications, low pricing, and great stability. Their low intrinsic activity, on the other hand, limits their electrochemical applicability, which can be enhanced by creating active spots and flaws.
Carbon materials’ inherent activity can be improved by doping heteroatoms (N, S, and P). N doped carbon materials have been considered to be one of the most promising ORR (Oxygen Reduction Reaction) catalysts among numerous heteroatom possibilities for doping carbon materials.
Two popular methods for preparing N-doped carbon materials include roasting organic materials with high N content after carbonization and employing NH3 in the biomass carbonization process. The former is risk-free and dependable, whereas the latter needs many processes to remove the remaining metal and templates.
Moreover, toxic HF solution and strong alkaline were employed to remove the template, which is exceedingly harmful. As a result, properly activating N-doped carbon in the process of biomass carbonization without raising the complexity of following treatments or equipment needs is a big scientific issue.
The current study not only establishes a simple and practicable green bridge for the conversion of wasted biomass into N-doped biochar catalysts for ORR and its proper implementation in the energy area but also provides a green and ecologically friendly way for reducing the environmental load.
Methodology
Buttonwood that had deteriorated for more than seven years was collected as a preliminary step. Ultrasonic cleaning was performed thrice using deionized water (DIW) and ethanol.
The washed RW was then boiled in DIW for 24 hours, with the DIW being refilled every 2, 4, 8, 14, and 22 hours. To eliminate moisture from the cooked RW, it was freeze-dried.
In the third stage, 20 g of NH4Cl was dissolved in 40 mL of DIW to make a supersaturated NH4Cl solution, which was then mixed with 0.5 g of RW and maintained in a boiling bath at 50 °C for 48 hours before being freeze-dried.
Finally, carbonization was carried out in a tube furnace at 1000 °C for 3 hours in an Ar atmosphere with a heating rate of 5 °C min-1. The carbonization temperature for NRW-900 and NRW-1100 was varied, but the preparation method was the same.
Without immersing in a supersaturated NH4Cl solution, RW-1000 was made.
SEM, TEM, N2 adsorption-desorption isotherms, XRD study, XPS, and Raman spectroscopy were among the techniques utilized.
After 30 minutes of ultrasonic vibration, the catalyst ink was created, and the long-term stability of the NRW-1000 and Pt/C catalysts were assessed by RDE in an O2-saturated 0.1 M KOH solution with a rotating speed of 400 rpm at a constant voltage of –0.5 V (vs. Ag/AgCl).
CV from 0.2 to –0.7V (vs. Ag/AgCl) in O2-saturated 0.1 M KOH solution with a scanning speed of 250 mV s–1 was used to assess the catalyst’s endurance.
NRW-1000 ink and Pt/C ink were equally coated on a 100-mesh stainless net pressed with a waterproof and breathable membrane (the catalyst loading capacity was 1.2 mg cm–2), which served as the cathode of the ZAB; the electrolyte was 6 M KOH with 0.2 M ZnCl2 aqueous solution.
The anode was a Zn sheet with a thickness of 3 mm. The LAND-CT2001A was used to evaluate the battery discharge performance in an atmospheric setting. A CHI 760E workstation was used to process the polarization data.
Using a total area of 1×4 cm2 and an average catalyst loading of 1.2 mg cm–2, the flexible ZAB was constructed with a Zn sheet as anode, gel-electrolyte as solid electrolyte, and NRW-1000 and Pt/C loaded on stainless mesh as the cathode.
The anode was a Zn sheet with a diameter of 16 mm and a thickness of 0.5 mm. As a solid electrolyte, the gel electrolyte created above was sliced into a diameter of 18 mm and a thickness of 1.5 mm.
The cathode area was 14 mm in diameter, with an average catalyst loading of 1.2 mg cm–2 (NRW-1000). The aforementioned electrode materials were used to manufacture the model 2032 button-type ZAB.
Results
Figure 1 depicts a schematic of the NRW-1000 preparation process. In the beginning, a natural RW of tung tree was selected as the raw material. Next, the washed RW was immersed in a saturated NH4Cl solution for 24 hours in a water bath (50 °C).
The NRW-1000 with 3D self-supporting structure was created by pyrolyzing NH4Cl @ RW in a tube furnace under an Ar environment (1000 °C, 3 hours) as the next step. Finally, three various varieties of ZAB (main, flexible, and button) were built using the NRW-1000.
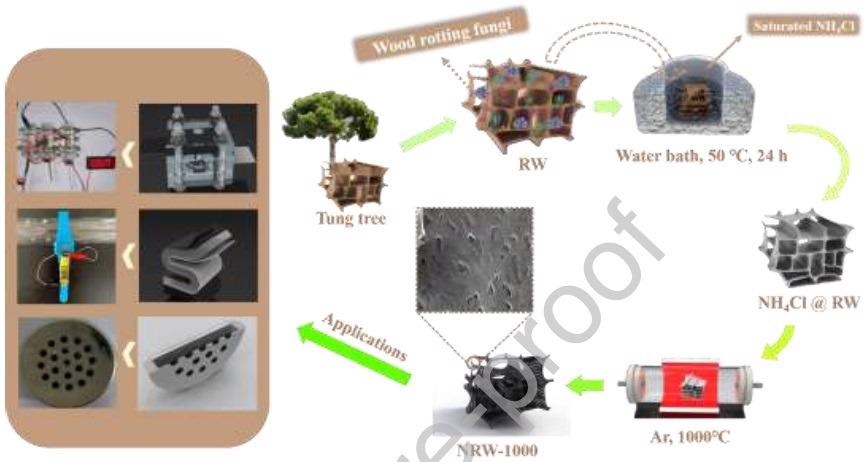
Figure 1: Schematic diagram of the preparation and applications of NRW-1000. Image Credit: Han, et al., 2022
Scanning electron microscopy (SEM) was used to examine the morphology of RW-based carbon compounds. The 3D structure that existed in virgin wood is still preserved after carbonization, as depicted in Figure 2a. RW-1000 exhibits irregular nanopores on the surface as a result of microbial degradation, as shown in Figure 2b.
Figure 2 c-e depicts the microstructure and morphology of NRW-1000. The NRW-1000 surface, illustrated in Figure 2c, not only has holes induced by microbial activity but also has a rough surface caused by NH3 corrosion from the breakdown of NH4Cl at high temperatures.
In NRW-1000, the in-situ exfoliated porous ultrathin graphene-like carbon nanosheets (GLCNs) are still adhered to the surface of the channel tube wall, as seen in Figure 2e, just like in NRW-900 and NRW-1100.
As shown in Figure 2f-i, the NRW-1000’s homogeneous distribution of N and O atoms was validated by element mapping.
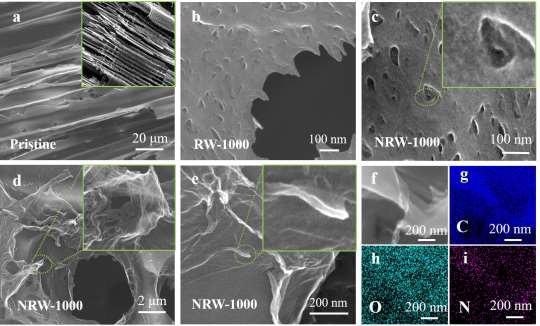
Figure 2. SEM images of RW-based samples. (a) Pristine wood, inside: carbonized wood; (b) Surface of RW-1000 (c) Surface and enlargement view of NRW-1000; (d) Surface peeled porous ultrathin GLCNs and enlarged view of NRW-1000; (e) The porous ultrathin GLCNs attract on the surface of NRW-1000; (f-i) Element mapping of NRW-1000. Image Credit: Han, et al., 2022
The crystal structure of RW and RW-based materials was studied using X-ray diffraction (XRD). The XRD pattern of pure RW, as shown in Figure 3a, reveals two distinct peaks at 2 θ of 15° and 22°, which correspond to the (101) and (200) interplanar of cellulose I, respectively.
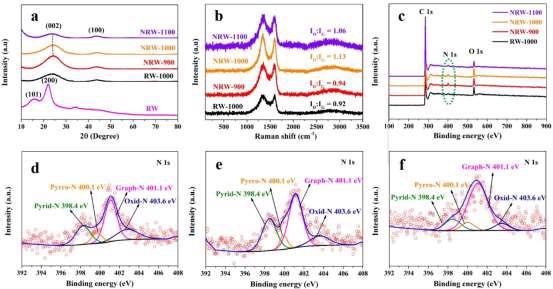
Figure 3. (a) XRD patterns; (b) Raman spectra; (c) XPS survey spectra of RW-based carbon materials; (d) N 1s of NRW-900; (e) N 1s of NRW-1000; (f) N 1s of NRW-1100. Image Credit: Han, et al., 2022
In the XRD patterns of RW-derived carbon materials, two widened peaks between 20°–30° and 40°–50°, corresponding to the carbon interplanar of (002) and (100), respectively, can be seen, indicating that amorphous regions exist in all RW-based carbon materials.
The degree of graphitization and defect of carbon materials were investigated using Raman spectroscopy. In carbon materials, the ID/IG value can be used to indicate the degree of flaws.
To investigate the chemical states of various atoms linked to C atoms in carbon compounds, X-ray photoelectron spectroscopy (XPS) studies were carried out.
C 1s, O 1s, and N 1s peaks can be found in the survey spectra of NRW-900, NRW-1000, and NRW-1100, but no N 1s peak can be seen in RW-1000, indicating that N doping in RW-based carbon materials may be achieved successfully using NH4Cl as an N source, as shown in Figure 3c.
Table 1 summarizes the results of quantitative XPS analyses.
The atomic O% of NRW-900, NRW-1000, and NRW-1100 are 6.50, 7.29, and 5.00, respectively, and they go through a rising and decreasing phase.
Table 1. Summary of quantitative XPS analyses. Source: Han, et al., 2022
|
C
(%) |
N
(%) |
O
(%) |
sp2/sp3 |
Pyrid-N
(%) |
Pyrro-N |
Graph-N
(%) |
Oxid-N
(%) |
NRW-900 |
90.35 |
3.15 |
6.5 |
4.17 |
24.44 |
9.95 |
45.25 |
20.36 |
NRW-1000 |
90.06 |
2.65 |
7.29 |
3.23 |
30.69 |
7.43 |
49.50 |
12.38 |
NRW-1100 |
92.79 |
2.21 |
5.00 |
5.16 |
10.33 |
5.91 |
74.67 |
9.09 |
Figure 4 shows transmission electron microscopy (TEM) and high-resolution TEM (HRTEM) images of RW-based carbon compounds.
In the HRTEM of the NRW-1000, many turbostratic graphite structures could be found, as shown in Figures 4b and c.
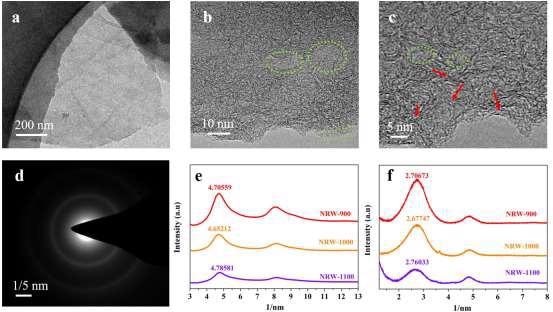
Figure 4. TEM and HRTEM images of NRW-1000. (a) TEM; (b and c) HRTEM, (dashed green circles: porous structure, red arrow: turbostratic graphite structures); (d) SAED; (e) The rotational average spectra corresponding to SAED pattern; (f) The transformation pattern of XRD. Image Credit: Han, et al., 2022
NRW-1000 has a Brunauer-Emmett-Teller (BET) surface area of 1917.83 m2 g–1, which is significantly higher than NRW-900 and NRW-1100.
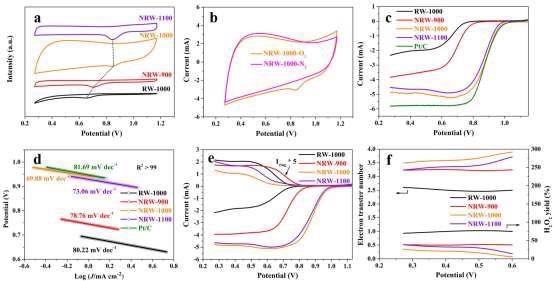
Figure 5. ORR performance: (a) CV curves of RW-based carbon materials; (b) CV curves of NRW-1000 in N2 and O2 saturated 0.1 M KOH aqueous solutions at scanning rate of 50 mV s–1; (c) LSV results at 1600 rpm; (d) Tafel slope; (e) RRDE at 1600 rpm; (f) H2O2 yield and electron transfer number. Image Credit: Han, et al., 2022
As demonstrated in Figure 5a, NRW-1000 has a higher positive oxygen reduction peak than other RW-based carbon materials, implying that it has the best ORR activity among RW-based carbon materials.
In contrast to NRW-1000’s featureless capacitive behavior in saturation N2 electrolyte, an apparent oxygen reduction peak is found in saturation O2 electrolyte, indicating that NRW-1000 has strong oxygen reduction activity as visible in Figure 5b.
The measured half-wave potentials of RW-1000, NRW-900, NRW-1000, NRW-1100, and Pt/C, as shown in Figure 5c are 0, 0.62, 0.87, 0.85, and 0.87 V, respectively, and the corresponding limiting currents are 2.32, 3.83, 4.85, 4.53, and 5.82 mA cm–2.
When compared to the other catalysts, the NRW-1000 has the highest ORR performance due to its larger positive half-wave potential and limited current density.
Determining the Tafel slopes from LSV curves was used to investigate the kinetics of ORR. The kinetics of NRW-1000 revealed the lowest Tafel slope, indicating that it has quicker kinetics.
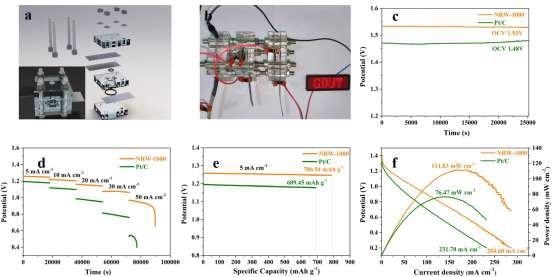
Figure 6. The assembled primary ZAB based on NRW-1000 and Pt/C (a) Schematic diagram of the primary ZAB; (b) Lighting-up the LED with the GDUT logo; (c) OCV kept for 7 h in a standard atmosphere; (d) Discharge curves at 5, 10, 20, 30, and 50 mA cm-2; (e) Specific capacity at a current density of 5 mA cm-2; (f) Polarization and power density curves. Image Credit: Han, et al., 2022
Figure 6a depicts the core ZAB assembly procedure as well as the location of each component. Three main ZABs based on NRW-1000 linked in series can light up the LED with the GDUT emblem as seen in Figure 6b.
The NRW-1000-based flexible ZAB was built, and its schematic design is shown in Figure 7a. The sole variation from the original ZAB is that PVA gel replaces the liquid electrolyte. The NRW-1000 maintains a constant OCV of 1.397 V at all bending angles, including 180°, demonstrating excellent flexibility and stability depicted in Figure 7b.
Wearable gadgets were given the flexible ZAB treatment, illustrated in Figure 7c. The specific capacity of NRW-1000 for 5 mA cm–2 was 710.23 mAh g–1Zn, with an energy density of 866.5 Wh kg–1Zn, which was greater than that of Pt/C, as shown in Figure 7d (597.61 mAh g–1Zn and 651.4 Wh kg–1Zn).
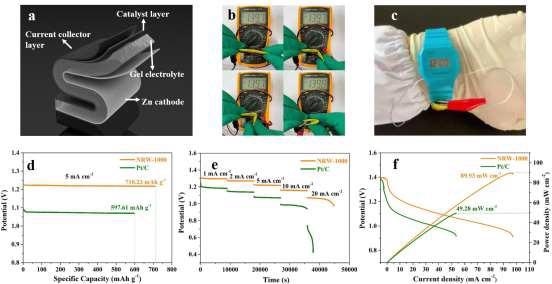
Figure 7. The assembled flexible ZAB based on NRW-1000 (a) Schematic diagram of the flexible ZAB; (b) Photographs of flexible ZAB bending at 0°, 30°, 90° and 180°; (c) ZAB applied to wearable devices; (d) Voltage diagrams at 1, 2, 5, 10, and 20 mA cm–2; (e) Polarization and power density curves of NRW-1000 and Pt/C. Image Credit: Han, et al., 2022
When the flexible ZAB based on NRW-1000 was employed as an electro-watch chain, it could be folded in any direction or angle without disrupting the watch's power supply, demonstrating exceptional wearability.
With an OCV of 1.423 V, a discharge voltage of 1.26, 1.23, 1.20, 1.13, and 1.03 V, and a power density of 58.52 mW cm–2, the button type ZAB based on NRW-1000 had a power density of 58.52 mW cm–2. It could be able to control a 3D-printed remote automobile.
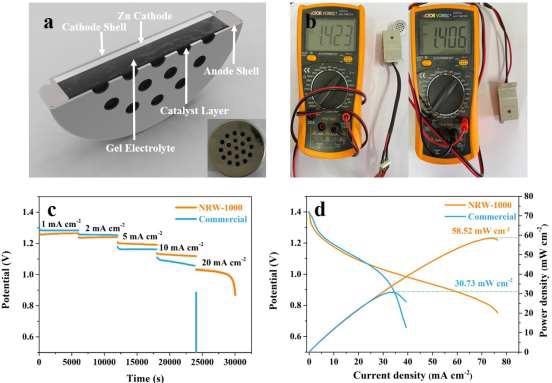
Figure 8. The assembled button ZAB based on NRW-1000 and commercial battery. (a) Schematic diagram of the button ZAB; (b) OCV of button ZAB based on NRW-1000 and commercial battery; (c) Voltage curves at different current densities of 1, 2, 5, 10, and 20 mA cm–2 and application in self-assembled cars; d) Polarization and power and density curves. Image Credit: Han, et al., 2022
Conclusions
In the paper, a facile and scalable technique for converting RW from bio-waste into N-doped carbon catalysts with better ORR performance is developed. The results show that the highest half-wave potential of the NRW-1000 is 0.87 V (vs. RHE), which is the same as that of Pt/C. (0.87 V).
The excellent results suggest that NRW-1000 might eventually replace Pt/C as a commercial catalyst. The study is intended to not only provide low-cost carbon-based cathode materials for commercial ZAB, but also to open up new paths for bio-waste utilization in a straightforward and realistic approach.
Journal Reference:
Han, S., Peng, S., Gao, Z., Sun, M., Cheng, G., Zhang, H., Su, X., Chen, M., and Yu, L. (2022) Green Bridge between Waste and Energy_Conversion the Rotten Wood into Cathode for Functional Zn-Air Battery. Electrochimica Acta. 140667 Available Online: https://www.sciencedirect.com/science/article/pii/S001346862200826X
References and Further Reading
et al. (2021) 3D N-doped ordered mesoporous carbon supported single-atom Fe-N-C catalysts with superior performance for oxygen reduction reaction and zinc-air battery. Applied Catalysis B: Environmental, 280, p. 119411. doi.org/10.1016/j.apcatb.2020.119411.
et al. (2022) Largescale defect-rich iron/nitrogen co-doped graphene-based materials as the excellent bifunctional electrocatalyst for liquid and flexible all-solid-state zinc-air batteries. Journal of Colloid and Interface Science, 607(P-2), pp. 1201−1214. doi.org/10.1016/j.jcis.2021.09.070.
Liu, Y., et al. (2021) Closely packed planar polyphthalocyanine iron/hierarchical three-dimensional graphene as an oxygen electrocatalyst for the ORR and OER, and zinc–air batteries. Sustainable Energy & Fuels, 5(20), pp. 5216−5226. doi.org/10.1039/D1SE00844G.
Chen, C. Y., et al. (2019) A Room‐Temperature Molten Hydrate Electrolyte for Rechargeable Zinc–Air Batteries. Advanced Energy Materials, 9(22), p. 1900196. doi.org/10.1002/aenm.201900196.
Yang, Z., et al. (2014) Electrochemical Energy Storage for Green Grid. Chemical Reviews, 111(5), pp. 3577−3613. doi.org/10.1021/cr100290v.
Li, Y & Dai, H (2014) Recent advances in zinc-air batteries. Chemical Society Reviews, 43(15) pp. 5257−5275. doi.org/10.1039/C4CS00015C.
Li, C. H., et al. (2019) Novel and multifunctional inorganic mixing salttemplated 2D ultrathin Fe/Co-N/S-carbon nanosheets as effectively bifunctional electrocatalysts for Zn-air batteries. Applied Catalysis B: Environmental, 241, pp. 95−103. doi.org/10.1016/j.apcatb.2018.09.024.
Doan, T. L. L., et al. (2021) Rational Engineering CoxOy Nanosheets via Phosphorous and Sulfur Dual-Coupling for Enhancing Water Splitting and Zn-Air Battery. Advanced Functional Materials, 31(10), p. 2007822. doi.org/10.1002/adfm.202007822.
Tang, K., et al. (2020) Inverse-opal-structured hybrids of N, S-codoped-carbon-confined Co9S8 nanoparticles as bifunctional oxygen electrocatalyst for on-chip all-solid-state rechargeable Zn-air batteries. Applied Catalysis B: Environmental, 260, p. 118209. doi.org/10.1016/j.apcatb.2019.118209.
Choi, S., et al. (2021) Highly efficient and stable bifunctional electrocatalysts with decoupled active sites for hydrogen evolution and oxygen reduction reactions. Applied Catalysis B: Environmental, 298, p. 120530. doi.org/10.1016/j.apcatb.2021.120530.
Wei Sun, F. W., et al. (2021) A rechargeable zinc-air battery based on zinc peroxide chemistry. Science, 371(6524), pp. 46−51. doi.org/10.1126/science.abb9554.
Xia, C., et al. (2018) A high-energy-density lithium-oxygen battery based on a reversible four-electron conversion to lithium oxide. Science, 361(6404), pp. 777−781. doi.org/10.1126/science.aas9343.
Ge, H., et al. (2020) Co4N nanoparticles encapsulated in N-doped carbon box as tri-functional catalyst for Zn-air battery and overall water splitting. Applied Catalysis B: Environmental, 275, p. 119104. doi.org/10.1016/j.apcatb.2020.119104.
Liu, S., et al. (2022) Nitrogen-Doped Carbon Polyhedrons Confined Fe–P Nanocrystals as High-Efficiency Bifunctional Catalysts for Aqueous Zn−CO2 Batteries, Small, 18(10), p. 2104965. doi.org/10.1002/smll.202104965.
Zhang, Y., et al. (2021) Enhancing bifunctionality of CoN nanowires by Mn doping for long-lasting Zn-air batteries. Science China Chemistry, 63, pp. 890−896. doi.org/10.1007/s11426-020-9739-2.
Cheng, F & Chen, J (2012) Metal-air batteries: from oxygen reduction electrochemistry to cathode catalysts. Chemical Society Reviews, 41(6), pp. 2172−2192. doi.org/10.1039/C1CS15228A.
Cong, G., et al. (2019) A high-rate and long-life organic–oxygen battery. Nature Materials, 18, pp. 390−396. doi.org/10.1038/s41563-019-0286-7.
Zhao, Y., et al. (2018) Few-layer graphdiyne doped with sp-hybridized nitrogen atoms at acetylenic sites for oxygen reduction electrocatalysis. Nature Chemistry, 10, pp. 924−931. doi.org/10.1038/s41557-018-0100-1.
Brodd, R J & Winter, M (2004) What Are Batteries, Fuel Cells, and Supercapacitors? Chemical Reviews, 104(10), pp. 4245−4269. https://doi.org/10.1021/cr020730k.
Chang, Y., et al. (2019) The fluorine-doped and defects engineered carbon nanosheets as advanced electrocatalysts for oxygen electroreduction. Applied Catalysis B: Environmental, 284, p. 119721. doi.org/10.1016/j.apcatb.2020.119721.
Xu, J., et al. (2019) Atomic Fe-Zn dual-metal sites for high-efficiency pH-universal oxygen reduction catalysis. Nano Research, 14, pp. 1374−1381. doi.org/10.1007/s12274-020-3186-x.
Seh, Z. W., et al. (2017) Combining theory and experiment in electrocatalysis: Insights into materials design. Science, 355(6321). doi.org/10.1126/science.aad4998.
Dai, L., et al. (2015) Metal-free catalysts for oxygen reduction reaction. Chemical Reviews, 115(11), pp. 4823−4892. doi.org/10.1021/cr5003563.
Masa, J., et al. (2015) On the Role of Metals in Nitrogen-Doped Carbon Electrocatalysts for Oxygen Reduction. Angewandte Chemie International Edition, 54(35), pp. 10102−10120. doi.org/10.1002/anie.201500569.
Gasteiger, H A & Marković, N M (2009) Just a Dream—or Future Reality? Science, 324(5923), pp. 48−49. doi.org/10.1126/science.1172083.
Liu, H., et al. (2022) Single palladium site in ordered porous heteroatom-doped carbon for high-performance alkaline hydrogen oxidation. Applied Catalysis B: Environmental, 306, p. 121029. doi.org/10.1016/j.apcatb.2021.121029.
Zhang, Y., et al. (2017) Ultrafine Metal Nanoparticles/N-Doped Porous Carbon Hybrids Coated on Carbon Fibers as Flexible and Binder-Free Water Splitting Catalysts. Advanced Energy Materials, 7(15), p. 1700220. doi.org/10.1002/aenm.201700220.
Xia, W., et al. (2016) Earth-Abundant Nanomaterials for Oxygen Reduction, Angewandte Chemie International Edition, 55(8), pp. 2650−2676. doi.org/10.1002/anie.201504830.
Wang, Z., et al. (2021) Understanding the Synergistic Effects of Cobalt Single Atoms and Small Nanoparticles: Enhancing Oxygen Reduction Reaction Catalytic Activity and Stability for Zinc-Air Batteries. Advanced Functional Materials, 31(45), p. 2104735. doi.org/10.1002/adfm.202104735.
Zhang, J., et al. (2021) Defect and Doping Co-Engineered Non-Metal Nanocarbon ORR Electrocatalyst. Nano-Micro Letters, 13, p. 65. doi.org/10.1007/s40820-020-00579-y.
Kong, F. T., et al. (2022) N-Doped Carbon Electrocatalyst: Marked ORR Activity in Acidic Media without the Contribution from Metal Sites? Angewandte Chemie International Edition, 61(15), p. e202116290. doi.org/10.1002/anie.202116290.
Wang, Y. P., et al. (2021) A gel-limiting strategy for large-scale fabrication of Fe-N-C single-atom ORR catalysts. Journal of Materials Chemistry A, 9(11), pp. 7137−7142. doi.org/10.1039/D0TA09228B.
Liang, J., et al. (2012) Sulfur and nitrogen dual-doped mesoporous graphene electrocatalyst for oxygen reduction with synergistically enhanced performance. Angewandte Chemie International Edition, 51(46) pp. 11496−11500. doi.org/10.1002/anie.201206720.
An, L., et al. (2018) Heterostructure-Promoted Oxygen Electrocatalysis Enables Rechargeable Zinc-Air Battery with Neutral Aqueous Electrolyte. Journal of the American Chemical Society, 140(50), pp. 17624−17631. doi.org/10.1021/jacs.8b09805.
Zheng, X. J., et al. (2019) N-, P-, and S-doped graphene-like carbon catalysts derived from onium salts with enhanced oxygen chemisorption for Zn-air battery cathodes. Applied Catalysis B: Environmental, 241, pp. 442−451. doi.org/10.1016/j.apcatb.2018.09.054.
Zhao, Z., et al. (2018) In Situ Activating Strategy to Significantly Boost Oxygen Electrocatalysis of Commercial Carbon Cloth for Flexible and Rechargeable Zn-Air Batteries. Advanced Science, 5(12), p. 1800760. doi.org/10.1002/advs.201800760.
Li, H., et al. (2021) N, F and S doped carbon nanofibers generated from electrospun polymerized ionic liquids for metal-free bifunctional oxygen electrocatalysis. Electrochimica Acta, 377, p. 138089. doi.org/10.1016/j.electacta.2021.138089.
Yang, D. S., et al. (2012) Phosphorus-doped ordered mesoporous carbons with different lengths as efficient metal-free electrocatalysts for oxygen reduction reaction in alkaline media. Journal of the American Chemical Society, 134(39), pp. 16127−16130. doi.org/10.1021/ja306376s.
Lei, W., et al. (2018) Two-Dimensional Phosphorus-Doped Carbon Nanosheets with Tunable Porosity for Oxygen Reactions in Zinc-Air Batteries. ACS Catalysis, 8(3), pp. 2464−2472. doi.org/10.1021/acscatal.7b02739.
Wang, Y., et al. (2021) Large-scale defect-engineering tailored tri-doped graphene as a metal-free bifunctional catalyst for superior electrocatalytic oxygen reaction in rechargeable Zn-air battery. Applied Catalysis B: Environmental, 285, p. 119811. doi.org/10.1016/j.apcatb.2020.119811.
Pampel, J & Fellinger, T -P (2016) Opening of Bottleneck Pores for the Improvement of Nitrogen Doped Carbon Electrocatalysts. Advanced Energy Materials, 6(8), p. 1502389. doi.org/10.1002/aenm.201502389.
Kuanping Gong, F. D., et al. (2009) Nitrogen-Doped Carbon Nanotube Arrays with High Electrocatalytic Activity for Oxygen Reduction. Science, 323(5915), pp. 760−764. doi.org/10.1126/science.1168049.
Wei, W., et al. (2014) Nitrogen-doped carbon nanosheets with size-defined mesopores as highly efficient metal-free catalyst for the oxygen reduction reaction. Angewandte Chemie International Edition, 53(6), pp. 1570−1574. doi.org/10.1002/anie.201307319.
Wang, C., et al. (2021) Novel space-confinement synthesis of two-dimensional Fe, N-codoped graphene bifunctional oxygen electrocatalyst for rechargeable air-cathode, Chemical Engineering Journal, 411, p. 128492. doi.org/10.1016/j.cej.2021.128492.
Pei, Z., et al. (2017) Texturing in situ: N,S-enriched hierarchically porous carbon as a highly active reversible oxygen electrocatalyst. Energy Environmental Science, 10(3), pp. 742−749. doi.org/10.1039/C6EE03265F.
Peng, X., et al. (2019) Hierachically Porous Carbon Plates Derived from Wood as Bifunctional ORR/OER Electrodes. Advanced Materials, 31(16), p. e1900341. doi.org/10.1002/adma.201900341.
Liu, Q., et al. (2016) Scalable Fabrication of Nanoporous Carbon Fiber Films as Bifunctional Catalytic Electrodes for Flexible Zn-Air Batteries. Advanced Materials, 28(15), pp. 3000−3006. doi.org/10.1002/adma.201506112.
Liu, Y., et al. (2020) Defect-Rich, Graphenelike Carbon Sheets Derived from Biomass as Efficient Electrocatalysts for Rechargeable Zinc-Air Batteries. ACS Sustainable Chemistry & Engineering, 8(7), pp. 2981−2989. doi.org/10.1021/acssuschemeng.9b07621.
Liu, Z., et al. (2017) In Situ Exfoliated, Edge-Rich, Oxygen-Functionalized Graphene from Carbon Fibers for Oxygen Electrocatalysis. Advanced Materials, 29(18), p. 1606207. doi.org/10.1002/adma.201606207.
Xiao, X., et al. (2020) Robust template-activator cooperated pyrolysis enabling hierarchically porous honeycombed defective carbon as highly-efficient metal-free bifunctional electrocatalyst for Zn-air batteries. Applied Catalysis B: Environmental, 265, p. 118603. doi.org/10.1016/j.apcatb.2020.118603.
Liu, L., et al. (2018) N-doped porous carbon nanosheets as pH-universal ORR electrocatalyst in various fuel cell devices. Nano Energy, 49, pp. 393−402. doi.org/10.1016/j.nanoen.2018.04.061.
He, Z. M., et al. (2022) Defective porous carbon microrods derived from fullerenes (C70) as high-performance electrocatalysts for the oxygen reduction reaction. Nanoscale, 14(2), pp. 473−481. doi.org/10.1039/D1NR07198J.
Hu, C., et al. (2022) A telluride-doped porous carbon as highly efficient bifunctional catalyst for rechargeable Zn-air batteries. Electrochimica Acta, 404, p. 139606. doi.org/10.1016/j.electacta.2021.139606.
Xiong, W., et al. (2020) Nitrogen-doped carbon nanotubes as a highly active metal-free catalyst for nitrobenzene hydrogenation. Applied Catalysis B: Environmental, 260, p. 118105. doi.org/10.1016/j.apcatb.2019.118105.
Zhang, X. R., et al. (2020) Revealing the dependence of active site configuration of N doped and N, S-co-doped carbon nanospheres on six-membered heterocyclic precursors for oxygen reduction reaction. Journal of Catalysis, 389, pp. 677−689. doi.org/10.1016/j.jcat.2020.07.003.
Fan, L. -Z., et al. (2018) Effects of the functional groups on the electrochemical properties of ordered porous carbon for supercapacitors. Electrochimica Acta, 105, pp. 299−304. doi.org/10.1016/j.electacta.2013.04.137.
Shinde, S. S., et al. (2018) Unveiling dual-linkage 3D hexaiminobenzene metal–organic frameworks towards long-lasting advanced reversible Zn–air batteries. Energy & Environmental Science, 12(2), pp. 727−738. doi.org/10.1039/C8EE02679C.
Ding, R., et al. (2020) Facile grafting strategy synthesis of single-atom electrocatalyst with enhanced ORR performance. Nano Research, 13, pp. 1519−1526. doi.org/10.1007/s12274-020-2768-y.
Hu, C. G., et al. (2021) Carbon-based metal-free electrocatalysts: from oxygen reduction to multifunctional electrocatalysis. Chemical Society Reviews, 50(21) pp. 11785−12260. doi.org/10.1039/D1CS00219H.
Huang, S., et al. (2017) N-, O-, and S-Tridoped Carbon-Encapsulated Co9S8 Nanomaterials: Efficient Bifunctional Electrocatalysts for Overall Water Splitting. Advanced Functional Materials, 27(17), p. 1606585. doi.org/10.1002/adfm.201606585.
Wollbrink, A., et al. (2016) Amorphous, turbostratic and crystalline carbon membranes with hydrogen selectivity. Carbon, 106, pp. 93−105. doi.org/10.1016/j.carbon.2016.04.062.
Lai, Q., et al. (2020) Optimal Configuration of N-Doped Carbon Defects in 2D Turbostratic Carbon Nanomesh for Advanced Oxygen Reduction Electrocatalysis. Angewandte Chemie International Edition, 59(29), pp. 11999−12006. doi.org/10.1002/anie.202000936.
Ji, H., et al. (2020) Pyridinic and graphitic nitrogen-enriched carbon paper as a highly active bifunctional catalyst for Zn-air batteries. Electrochimica Acta, 334, p. 135562. doi.org/10.1016/j.electacta.2019.135562.
Yang, M., et al. (2022) Integration of partially phosphatized bimetal centers into trifunctional catalyst for high-performance hydrogen production and flexible Zn-air battery. Science China Materials, 65, pp. 1176−1186. doi.org/10.1007/s40843-021-1902-2.