Human life depends on an efficient and long-term agricultural production system. Agricultural productivity, however, is suffering from severe environmental deterioration. Many new scientific and technical innovations have evolved to supply the human demand for food, such as new procedures (gene editing) and advanced materials (nanomaterials). This has been explored in the journal Crop Design.
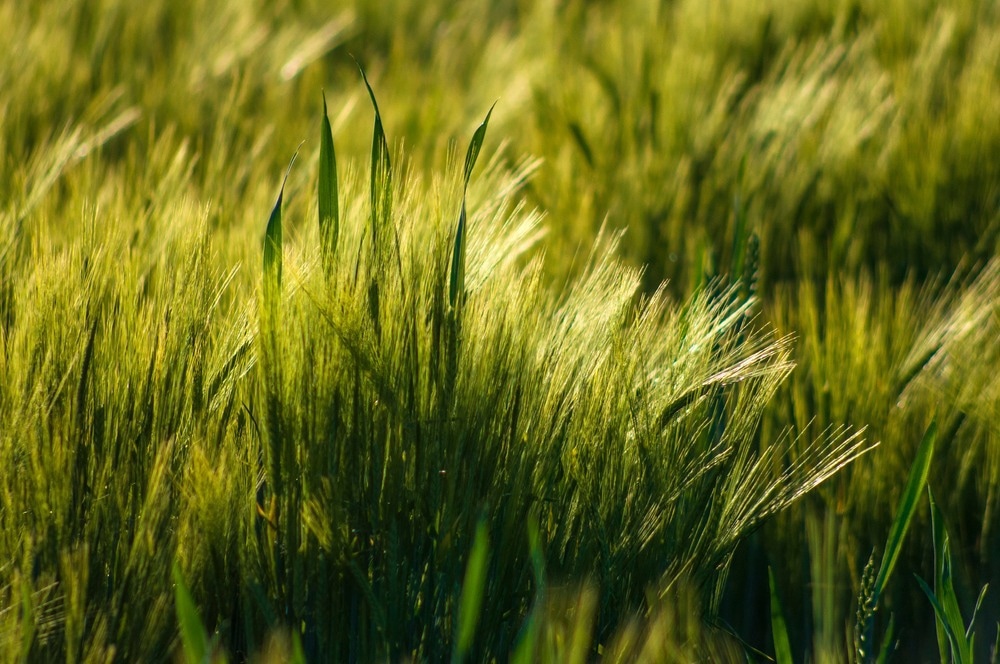
Study: Opportunities for graphene, single-walled and multi-walled carbon nanotube applications in agriculture: A review. Image Credit: FotoGam/Shutterstock.com
Nanomaterials are widely employed in electrical, mechanical, energy, and biomedical applications. Researchers discovered that nanomaterials could be absorbed by plants, transported to various tissues and organs, improved seed germination rates, and promoted plant development.
Nanomaterials can also be utilized to create sensors that collect real-time data on plant physiological conditions to improve plants’ ability to respond to environmental stresses and increase agricultural and forest output. Metals, metal oxides, carbon nanoparticles, and polymers are the most common nanomaterials, with carbon nanomaterials attracting the most attention from researchers due to their unique chemical characteristics, structures, and low toxicity.
Single-walled carbon nanotubes, multi-walled carbon nanotubes, graphene, carbon nanohorns, carbon nano-onions, fullerenes, and carbon nano quantum dots are the most common carbon nanomaterials discovered so far. Carbon nanoparticles can infiltrate plant cells and deliver DNA vectors, as well as affect seed germination and plant growth.
Graphene is a single-walled carbon nanotube made out of a two-dimensional one-atom-thick sheet of carbon coiled into a cylinder. Multi-walled carbon nanotubes wrapped into cylinders inside other carbon cylinders can be used to make multiple graphene sheets. They all have a large specific surface area and may be engineered to accommodate a wide range of oxygen-containing functional groups, such as carboxyl groups.
Carbon nanoparticles in agriculture have received a lot of attention in recent years. This paper summarizes the nanoparticle applications in single- and multi-walled nanotubes and graphene in crop breeding. Graphene’s impacts on plant growth, water, fertilizer, and pesticide use, environmental stress, the plant-graphene interaction mechanism, and its environmental effects were also studied. This study serves as a resource for key carbon nanomaterial uses in agriculture.
Methodology
The goal of agricultural genetic engineering is to create pathogen-resistant and high-yielding crops. Plant cell walls, on the other hand, act as a barrier to foreign biomolecule delivery. Conventional gene delivery technologies, such as Agrobacterium-mediated genetic transformation and gene guns, are ineffective, cause plant tissue damage and only work in a few plant species and genotypes.
Nanoparticles having a diameter of less than 50 nm are potential materials for transporting biomolecules into plant cells for genetic engineering to develop crops with desirable characteristics.
SWCNTs (single-walled carbon nanotubes) have a diameter of around 1 nm and can readily pass through the plant cell wall barrier. Researchers have devised a way to graft DNA onto SWCNTs via electrostatic attraction as a result of this characteristic.
To carry a net positive charge, carboxylated single-walled carbon nanotubes (COOH-SWCNTs) were covalently altered with poly-ethylenimine (PEI). After that, negatively charged phosphate groups on plasmid DNA vectors are incubated with positively charged PEI–COOH–SWCNTs.
This cargo tool was recently used to accomplish high-efficiency green fluorescent protein (GFP) gene delivery as well as a transient expression without transgene integration in mature leaf and protoplast cells of both dicot and monocot plants, such as Nicotiana benthamiana, Gossypium hirsutum, Eruca sativa, and Triticum aestivum.
Because of their bigger particle size, multi-walled carbon nanotubes (MWCNTs) are less efficient in delivering DNA into plant cells than SWCNTs. The benefits of multi-walled carbon nanotubes in plant gene transfer were demonstrated in this study.
In living cells, graphene oxide and poly-ethylenimine (PEI) conjugates demonstrated a high transfection efficiency of enhanced green fluorescent protein (EGFP). The use of graphene oxide substrates to preconcentrate PEI/pDNA complexes resulted in effective gene transport and gene transfection in a variety of cell lines, involving stem cell research and tissue engineering.
Incorporating foreign vectors of DNA, mRNA, RNAi, or CRISPR/Cas9 into the crop host genome using graphene-mediated transformation technologies in agriculture has the potential to impact crop genetic manipulation in the future.
Graphene biosensors, impacts of graphene on plant physiology, seedling root growth, seed germination, plant biomass, and food quality, as well as water, fertilizer, and pesticide consumption efficiency in agriculture, are all areas of current research on graphene’s influence on plants.
The interaction of biomaterials and graphene may be used as a nanosensor platform for monitoring bacterial development, according to a recent breakthrough. These findings suggest that graphene might be utilized as a biosensor to swiftly sense and gather target desired trait information in crops, thereby speeding up the breeding selection process.
According to a new study, graphene can make the Asian corn borer “fatter” while also shortening its lifespan. In the future, this type of graphene-based pesticide delivery device might be used to protect plants. In a recent study, graphene was utilized to treat 48 plant types to see how it affected root development.
C atoms make up the majority of graphene, and its surface is rich in oxygen-containing functional groups like carboxyl (-COOH) and hydroxyl (-OH). At low concentrations, these functional groups and cation-π interactions in graphene can attract cations (e.g., NH4+ and K+) in the soil, nutrient supply for plant root growth.
Results
For sensitive consumers, the usage of graphene in food crops might pose a safety risk. Researchers employed 14C-labeled graphene to study the uptake, distribution, conversion, and depuration of graphene in rice, discovering that graphene may be transferred swiftly from roots to shoots and leaves. 14C-labeled graphene may get through cell walls and membranes into the chloroplast of leaves, where it reacts with OH−, causing graphene to degrade via mineralization into 14CO2.
Although rice may absorb graphene, long-cycle experiments revealed that the buildup of graphene in stems and leaves vanished 15 days after exposure to graphene, and also no graphene remained in harvested rice. In addition, it was discovered that microorganisms in the soil environment might destroy graphene.
Because the polycyclic structure of graphene is comparable to that of lignin and polycyclic aromatic hydrocarbons, lignin enzymes released by certain fungi can break down and destroy graphene. This research might be useful in addressing public concerns about the safety of using engineered graphene in agricultural production.
Conclusion
Carbon nanomaterial-based innovations might help agriculture systems become more sustainable, efficient, and robust. Carbon nanomaterials in agriculture are becoming more widely used, and the fundamental knowledge of carbon nanomaterial-plant interactions will continue to improve.
Another area where carbon nanomaterials may be used in agriculture is to improve biomolecule and agrochemical delivery efficiency, including delivery timing, location, dosage, and shape. As a result, it is possible that carbon nanomaterials, such as single-walled carbon nanotubes, multi-walled carbon nanotubes, and graphene, will be used to create “smart plants” in the future.
Journal Reference:
Chen, Z., Zhao, J., Cao, J., Zhao, Y., Huang, J., Zheng, Z., Li, W., Jiang, S., Qiao, J., Xing, B., Zhang, J. (2022). Opportunities for graphene, single-walled and multi-walled carbon nanotube applications in agriculture: A review. Crop Design, 1(1), p.100006. Available Online: https://www.sciencedirect.com/science/article/pii/S2772899422000064
References and Further Reading
- Willett, W., et al. (2019) Food in the Anthropocene: the EAT-Lancet Commission on healthy diets from sustainable food systems Epub 2019/01/21. Lancet, 393(10170), pp. 447–492. doi.org/10.1016/S0140-6736(18)31788-4.
- Lowry, G. V. et al. (2019) Opportunities and challenges for nanotechnology in the agri-tech revolution. Nature Nanotechnology, 14(6), pp. 517–522. doi.org/10.1016/S0140-6736(18)31788-4.
- Klarich, K. L., et al. (2017) Occurrence of neonicotinoid insecticides in finished drinking water and fate during drinking water treatment. Environmental Science & Technology Letters, 4, pp. 168–173. doi.org/10.1021/acs.estlett.7b00081.
- Beketov, M. A., et al. (2013) Pesticides reduce regional biodiversity of stream invertebrates. Proceedings of the National Academy of Sciences of the United States of America, 110(27), pp. 11039–11043, doi.org/10.1073/pnas.1305618110.
- Kah, M., et al. (2019) Nano-enabled strategies to enhance crop nutrition and protection. Nature Nanotechnology, 14(6), pp. 532–540. doi.org/10.1038/s41565-019-0439-5.
- Liu, X. H., et al. (2012) In situ atomic-scale imaging of electrochemical lithiation in silicon. Nature Nanotechnology, 7(11), pp. 749–756. doi.org/10.1038/nnano.2012.170.
- Shi, W., et al. (2016) Mechanics of an asymmetric hard-soft lamellar nanomaterial. ACS Nano, 10(2), pp. 2054–2062. doi.org/10.1021/acsnano.5b06215.
- Pang, H., et al. (2012) Facile synthesis of porous ZnO-NiO composite micropolyhedrons and their application for high power supercapacitor electrode materials. Dalton Transactions, 41(43), pp. 13284–13291. doi.org/10.1039/c2dt31916k.
- Valentini, F., et al. (2013) Carbon nanostructured materials for applications in nano-medicine, cultural heritage, and electrochemical biosensors. Analytical and Bioanalytical Chemistry, 405(2–3), pp. 451–465. doi.org/10.1007/s00216-012-6351-6.
- Zuverza-Mena, N., et al. (2017) Exposure of engineered nanomaterials to plants: insights into the physiological and biochemical responses-A review. Plant Physiology and Biochemistry, 110, pp. 236–264. doi.org/10.1016/j.plaphy.2016.05.037.
- Canas, J. E. et al. (2008) Effects of functionalized and nonfunctionalized single-walled carbon nanotubes on root elongation of select crop species. Environmental Toxicology and Chemistry, 27(9), pp. 1922–1931. doi.org/10.1897/08-117.1.
- Giraldo, J. P., et al. (2019) Nanobiotechnology approaches for engineering smart plant sensors. Nature Nanotechnology, 14(6), pp. 541–553. doi.org/10.1038/s41565-019-0470-6.
- Mukherjee, A., et al. (2016) Carbon nanomaterials in agriculture: a critical review. Frontiers in Plant Science, 7, p. 172. doi.org/10.3389/fpls.2016.00172.
- Chichiricco, G & Poma, A (2015) Penetration and toxicity of nanomaterials in higher plants. Nanomaterials, 5(2), pp. 851–873. doi.org/10.3390/nano5020851.
- Nair, R., et al. (2012) Effect of carbon nanomaterials on the germination and growth of rice plants. Journal of Nanoscience and Nanotechnology, 12(3), pp. 2212–2220. doi.org/10.1166/jnn.2012.5775.
- Yan, S., et al. (2013) Single-walled carbon nanotubes selectively influence maize root tissue development accompanied by the change in the related gene expression. The Journal of Hazardous Materials, 246–247, pp. 110–118. doi.org/10.1016/j.jhazmat.2012.12.013.
- Zhang, X., et al. (2022) The effects of graphene-family nanomaterials on plant growth: a review. Nanomaterials, 12(6). doi.org/10.3390/nano12060936.
- Eatemadi, A., et al. (2014) Carbon nanotubes: properties, synthesis, purification, and medical applications. Nanoscale Research Letters, 9(1), p. 393. doi.org/10.1186/1556-276X-9-393.
- Chae, S., et al. (2020) Anomalous restoration of sp(2) hybridization in graphene functionalization. Nanoscale, 12(25), pp. 13351–13359. doi.org/10.1039/d0nr03422c.
- Alazmi, A., et al. (2016) A process to enhance the specific surface area and capacitance of hydrothermally reduced graphene oxide. Nanoscale, 8(41), pp. 17782–17787. doi.org/10.1039/c6nr04426c.
- Nyaboga, E., et al. (2014) Agrobacterium-mediated genetic transformation of yam (Dioscorea rotundata): an important tool for functional study of genes and crop improvement. Frontiers in Plant Science, 5, p. 463. doi.org/10.3389/fpls.2014.00463.
- Cunningham, F. J., et al. (2018) Nanoparticle-mediated delivery towards advancing plant genetic engineering. Trends Biotechnology, 36(9), pp. 882–897. doi.org/10.1016/j.tibtech.2018.03.009.
- Al-Qattan, M. N., et al. (2018) Molecular dynamics simulation strategies for designing carbon-nanotube-based targeted drug delivery. Drug Discovery Today, 23(2), pp. 235–250. doi.org/10.1016/j.drudis.2017.10.002.
- Demirer, G. S., et al. (2019) High aspect ratio nanomaterials enable delivery of functional genetic material without DNA integration in mature plants. Nature Nanotechnology, 14(5), pp. 456–464. doi.org/10.1038/s41565-019-0382-5.
- Kwak, S. Y., et al. (2019) Chloroplast-selective gene delivery and expression in planta using chitosan-complexed single-walled carbon nanotube carriers. Nature Nanotechnology, 14(5), pp. 447–455. doi.org/10.1038/s41565-019-0375-4.
- Zhang, H., et al. (2019) DNA nanostructures coordinate gene silencing in mature plants. Proceedings of the National Academy of Sciences of the United States of America, 116(15), pp. 7543–7548. doi.org/10.1073/pnas.1818290116.
- Burlaka, O. M., et al. (2015) Plant genetic transformation using carbon nanotubes for DNA delivery. Cytology and Genetics, 49(6), pp. 3–12. doi.org/10.3103/S009545271506002X.
- Serag, M. F., et al. (2011) Trafficking and subcellular localization of multiwalled carbon nanotubes in plant cells. ACS Nano, 5(1), pp. 493–499. doi.org/10.1021/nn102344t.
- Mirzaie, V., et al. (2020) Nano-graphene oxide-supported APTES-spermine, as gene delivery system, for transfection of pEGFP-p53 into breast cancer cell lines. Drug Design, Development and Therapy, 14, pp. 3087–3097. doi.org/10.2147/DDDT.S251005.
- Liu, Y., et al. (2021) A novel graphene quantum dot-based mRNA delivery platform. ChemistryOpen, 10(7), pp. 666–671. doi.org/10.1002/open.202000200.
- Di Santo, R., et al. (2019) Microfluidic manufacturing of surface-functionalized graphene oxide nanoflakes for gene delivery. Nanoscale, 11(6), pp. 2733–2741. doi.org/10.1039/c8nr09245a.
- Teimouri, M., et al. (2016) Graphene oxide-cationic polymer conjugates: synthesis and application as gene delivery vectors. Plasmid, 84–85, pp. 51–60. doi.org/10.1016/j.plasmid.2016.03.002.
- Li, K., et al. (2014) Patterned substrates of nano-graphene oxide mediating highly localized and efficient gene delivery. ACS Applied Materials & Interfaces, 6(8), pp. 5900–5907. doi.org/10.1021/am5008134.
- Demirer, G. S., et al. (2021) Nanotechnology to advance CRISPR-Cas genetic engineering of plants. Nature Nanotechnology, 16(3), pp. 243–250. doi.org/10.1038/s41565-021-00854-y.
- Demirer, G. S., et al. (2019) Carbon nanotube–mediated DNA delivery without transgene integration in intact plants. Nature Protocols, 14(10), pp. 2954–2971. doi.org/10.1038/s41596-019-0208-9.
- Landry, M P & Mitter, N (2019) How nanocarriers delivering cargos in plants can change the GMO landscape. Nature Nanotechnology, 14(6), pp. 512–514. doi.org/10.1038/s41565-019-0463-5.
- Zhang, M., et al. (2015) Effects of graphene on seed germination and seedling growth. Journal of Nanoparticle Research, 17(2), p. 78. doi.org/10.1007/s11051-015-2885-9.
- Hoseini-Ghahfarokhi, M., et al. (2020) Applications of graphene and graphene oxide in smart drug/gene delivery: is the world still flat? International Journal of Nanomedicine, 15, pp. 9469–9496. doi.org/10.2147/IJN.S265876.
- Mandal, D., et al. (2019) DNA supported graphene quantum dots for Ag ion sensing. Nanotechnology, 30(25), Article, p. 255501. doi.org/10.1088/1361-6528/ab084c.
- Begum, P., et al. (2011) Graphene phytotoxicity in the seedling stage of cabbage, tomato, red spinach, and lettuce. Carbon, 49(12), pp. 3907–3919. doi.org/10.1016/j.carbon.2011.05.029.
- He, Y. J., et al. (2018) Graphene oxide as a water transporter promoting germination of plants in soil. Nano Research, 11(4), pp. 1928–1937. doi.org/10.1007/s12274-017-1810-1
- Liu, S., et al. (2015) Effects of graphene on germination and seedling morphology in rice. Journal of Nanoscience and Nanotechnology, 15(4), pp. 2695–2701. doi.org/10.1166/jnn.2015.9254.
- Zhang, M., et al. (2014) Slow-release fertilizer encapsulated by graphene oxide films. The Chemical Engineering Journal, 255, pp. 107–113. doi.org/10.1007/978-3-642-40371-2_15.
- Pandey, R R & Chusuei, C C (2021) Carbon nanotubes, graphene, and carbon dots as electrochemical biosensing composites. Molecules, 26(21). doi.org/10.3390/molecules26216674 .
- Yim, Y., et al. (2021) Graphene oxide-based fluorescent biosensors and their biomedical applications in diagnosis and drug discovery. Chemical Communications, 57(77), pp. 9820–9833. doi.org/10.1039/d1cc02157e.
- Shahriari, S., et al. (2021) Graphene and graphene oxide as a support for biomolecules in the development of biosensors. Nanotechnology, Science and Applications, 14, pp. 197–220. doi.org/10.2147/NSA.S334487.
- Bharath, G., et al. (2015) Enzymatic electrochemical glucose biosensors by mesoporous 1D hydroxyapatite-on-2D reduced graphene oxide. Journal of Materials Chemistry B, 3(7), pp. 1360–1370. doi.org/10.1039/c4tb01651c.
- Ortiz-Riano, E. J., et al. (2020) Microwell plates coated with graphene oxide enable advantageous real-time immunosensing platform. Biosensors and Bioelectronics, 165, Article, p. 112319. doi.org/10.1016/j.bios.2020.112319.
- Mohanty, N & Berry, V (2008) Graphene-based single-bacterium resolution biodevice and DNA transistor: interfacing graphene derivatives with nanoscale and microscale biocomponents. Nano Letters, 8(12), pp. 4469–4476. doi.org/10.1021/nl802412n.
- Huang, Y., et al. (2011) Graphene-based biosensors for detection of bacteria and their metabolic activities. Journal of Materials Chemistry, 21(33), pp. 12358–12362. doi.org/10.1039/C1JM11436K.
- Mannoor, M. S., et al. (2012) Graphene-based wireless bacteria detection on tooth enamel. Nature Communication, 3, p. 763. doi.org/10.1038/ncomms1767.
- Zhao, D., et al. (2020) Graphene oxide as an effective soil water retention agent can confer drought stress tolerance to Paeonia ostii without toxicity. Environmental Science & Technology, 54(13), pp. 8269–8279. doi.org/10.1021/acs.est.0c02040.
- Urso, J H & Gilbertson, L M (2018) Atom conversion efficiency: a new sustainability metric applied to nitrogen and phosphorus use in agriculture. ACS Sustainable Chemistry & Engineering, 6, pp. 4453–4463. doi.org/10.1021/acssuschemeng.7b03600%20 (2018).
- Kabiri, S., et al. (2017) Graphene oxide: a new carrier for slow release of plant micronutrients. ACS Applied Materials & Interfaces, 9(49), pp. 43325–43335. doi.org/10.1021/acsami.7b07890.
- Li, T., et al. (2019) Chitosan and graphene oxide nanocomposites as coatings for controlled-release fertilizer. Water Air & Soil Pollutions, 230(7). doi.org/10.1007/s11270-019-4173-2.
- Anjum, N. A., et al. (2013) Single-bilayer graphene oxide sheet tolerance and glutathione redox system significance assessment in faba bean (Vicia faba L). Journal of Nanoparticle Research, 15(7), p.1770. doi.org/10.1007/s11051-013-1770-7.
- Chakravarty, D., et al. (2015) Graphene quantum dots as enhanced plant growth regulators: effects on coriander and garlic plants. Journal of the Science of Food and Agriculture, pp. 2772–2778. doi.org/10.1002/jsfa.7106.
- Wang, X., et al. (2019) Graphene oxide as a pesticide delivery vector for enhancing acaricidal activity against spider mites. Colloids and Surfaces B: Biointerfaces, 173, pp. 632–638. doi.org/10.1016/j.colsurfb.2018.10.010.
- Wang, X., et al. (2019) Graphene oxide as a multifunctional synergist of insecticides against lepidopteran insect. Environmental Science: Nano, 6(1), pp. 75–84. doi.org/10.1039/C8EN00902C.
- Wang, X., et al. (2021) Phenotypic responses and potential genetic mechanism of lepidopteran insects under exposure to graphene oxide. Ecotoxicology and Environmental Safety, 228, Article, p. 113008. doi.org/10.1016/j.ecoenv.2021.113008.
- Wang, W., et al. (2003) Plant responses to drought, salinity and extreme temperatures: towards genetic engineering for stress tolerance. Planta, 218(1), pp. 1–14. doi.org/10.1007/s00425-003-1105-5.
- Savary, S., et al. (2012) Crop losses due to diseases and their implications for global food production losses and food security. Food Secure, 4(4), pp. 519–537. doi.org/10.1007/s12571-012-0200-5.
- Suzuki, N., et al. (2014) Abiotic and biotic stress combinations. New Phytologist, 203(1), pp. 32–43. doi.org/10.1111/nph.12797.
- Liu, Z., et al. (2020) Effects of graphene on the growth and development of Vicia faba L. International Journal of Wireless and Mobile Computing (IJWMC), 41(5), pp. 33–39.
- Gao, M., et al. (2020) Foliar graphene oxide treatment increases photosynthetic capacity and reduces oxidative stress in cadmium-stressed lettuce. Plant Physiology and Biochemistry, 154, pp. 287–294. doi.org/10.1016/j.plaphy.2020.06.021.
- Kumar, P., et al. (2019) Antibacterial properties of graphene-based nanomaterials. Nanomaterials, 9(5), doi.org/10.3390/nano9050737.
- He, Y., et al. (2018) Graphene oxide as an antimicrobial agent can extend the vase life of cut flowers. Nano Research, 11, pp. 6010–6022. doi.org/10.1007/s12274-018-2115-8.
- Wang, X., et al. (2019) Metabonomics-assisted label-free quantitative proteomic and transcriptomic analysis reveals novel insights into the antifungal effect of graphene oxide for controlling Fusarium graminearum. Environmental Science: Nano, International Journal of Environmental Research, 6(11), pp. 3401–3421. doi.org/10.1039/C9EN00981G.
- Gao, M., et al. (2020) Effects of foliar application of graphene oxide on cadmium uptake by lettuce. The Journal of Hazardous Materials, 398, Article, p. 122859. doi.org/10.1016/j.jhazmat.2020.122859.
- Hu, C., et al. (2018) Effect of graphene oxide on copper stress in Lemna minor L.: evaluating growth, biochemical responses, and nutrient uptake. The Journal of Hazardous Materials, 341, pp. 168–176. doi.org/10.1016/j.jhazmat.2017.07.061.
- Wang, Q., et al. (2019) Phytotoxicity of graphene family nanomaterials and its mechanisms: a review. Frontiers in Chemistry, 7, p. 292. doi.org/10.3389/fchem.2019.00292.
- Hu, X., et al. (2019) Effect of graphene on the growth and development of Raspberry tissue culture seedlings. New carbon material,34(5), pp.447–454.
- Xue, B., et al. (2019) Effects of graphene oxide on growth and physiological characteristics of tissue culture seedlings of Rubus corchorifoliuslf under salt stress SHANDONG FORESTRY. Science Technology, (4), pp. 23–28.
- Guo, X., et al. (2019) Effects of graphene on root morphology and biomass of Quinoa seedlings. Journal of Shanxi Agricultural Sciences, 47(8), pp. 1395–1398. doi.org/10.3969/j.issn.1002-2481.2019.08.22.
- Chen, Z., et al. (2021) Influence of graphene on the multiple metabolic pathways of Zea mays roots based on transcriptome analysis. PLoS One, 16(1), p. e0244856. doi.org/10.1371/journal.pone.0244856.
- Zhang, X., et al. (2021) Graphene oxide exhibited positive effects on the growth of Aloe vera L. Physiology and Molecular Biology of Plants, 27(4), pp. 815–824. doi.org/10.1007/s12298-021-00979-3.
- Guo, X., et al. (2021) Effects of graphene oxide on tomato growth in different stages. Physiology and Molecular Biology of Plants, 162, pp. 447–455. doi.org/10.1016/j.plaphy.2021.03.013.
- Zhang, X., et al. (2021) Effects of graphene on morphology, microstructure and transcriptomic profiling of Pinus tabuliformis Carr. Roots. PLoS One, 16(7), p. e0253812. doi.org/10.1371/journal.pone.0253812.
- Chen, Z., et al. (2022) Graphene-mediated antioxidant enzyme activity and respiration in plant roots. ACS Agricultural Science & Technology, doi.org/10.1021/acsagscitech.2c00074.
- Qiao, J., et al. (2017) Review of effects of carbon nano-materials on crop growth. Transactions of the Chinese Society of Agricultural Engineering, 33(2), pp. 170–178.
- Sui, Q., et al. (2019) Effect of combined application of graphene solution and fertilizer on soil nutrient loss. Journal of Soil and Water Conservation, 33(1), pp. 41–46. doi.org/10.1021/acsagscitech.2c00074.
- Zhao, G & Zhu, H (2020) Cation-pi interactions in graphene-containing systems for water treatment and beyond. Advance Materials, p. e1905756. doi.org/10.1002/adma.201905756.
- Lombi, E., et al. (2019) A One Health approach to managing the applications and implications of nanotechnologies in agriculture. Nature Nanotechnology, 14(6), pp. 523–531. doi.org/10.1038/s41565-019-0460-8.
- Huang, C., et al. (2018) Transformation of (14) C-labeled graphene to (14) CO2 in the shoots of a rice plant. Angewandte Chemie International Edition in English, 57(31), pp. 9759–9763. doi.org/10.1002/anie.201805099.
- Lalwani, G., et al. (2014) Enzymatic degradation of oxidized and reduced graphene nanoribbons by lignin peroxidase. Journal of Materials Chemistry B, 2(37), pp. 6354–6362. doi.org/10.1039/C4TB00976B.
- Qu, Y., et al. (2018) A novel environmental fate of graphene oxide: biodegradation by a bacterium Labrys sp. WJW to support growth. Water Research, 143, pp. 260–269. doi.org/10.1016/j.watres.2018.03.070.