The need to visualize the dynamic evolution of chemicals mixing and reacting at the microscale is increasing. There are a variety of applications for this visualisation; from controlling the syntheses of drugs in micro-flow channels to monitoring the uptake of drugs in live cell experiments.
The Spero microscope from Daylight Solutions is a one-of-a-kind microscope that enables the monitoring of microscale flow chemistries across large areas, at high spatial resolutions and at video rates in real time.
Spero Microscope
The Spero microscope uses tunable laser technology as its light source. It shines a narrow band of infrared (IR) laser light onto the sample. The light’s frequency can be tuned across the fingerprint mid-infrared spectral range to match the molecular absorption peaks in the target compounds, generating a functional group specific chemical contrast image at the detector.
The user has complete control of the excitation frequency of this illumination and simultaneous imaging of various chemistries at video frame rates within the same field-of-view is possible. Full infrared spectra can be obtained by tuning the laser source across the frequency range of interest.
Other infrared methods, such as Fourier Transform Infrared (FTIR) microspectroscopy, have not had great success in monitoring dynamic processes. Also, these methods are highly restricted in spatial resolution and dynamic response.
The Spero microscope is the only infrared imaging method with the ability to offer both dynamic and spatial chemical data in real time. The Spero microscope is the first and only infrared microscope to use a quantum cascade laser (QCL), which has been commercialized over the last 10 years.
The QCL source is five orders of magnitude brighter than the Globar source, which is generally used in FTIR instruments, and three orders of magnitude brighter than a synchrotron source. It has the ability to use large format, uncooled microbolometer array detectors and higher sensitivity. The detectors provide mid-IR detection with a better linear dynamic range, pixel densities of 480 x 480, and with read-out rates of 30 fps. They are highly reliable and don't require any cool-down time or cryogens.
Spero is well-suited for real-time measurements; where the spatial distribution of definite functional groups and chemical data are taken from dynamic processes. Applications include reaction monitoring, melting, diffusion studies, liquid-liquid interactions, microfluidics, flow chemistry, and crystallization and studies. Diverse applications such as affinity studies and the observation of drug responses in living cells can also be conducted.
Visualizing Chemistry and Flow within a Microfluidic System
How the HOD species are formed and distributed was visualized using the Spero microscope during deuteron/proton exchange between deuterium oxide and water streams inside a microfluidic cell.
The Spero's exceptional real-time imaging capabilities facilitate the observation of diffusion, flow dynamics, advection and reaction products at a full 30 fps video with a 1.4 µm per pixel resolution at the users' preferred infrared frequencies. Absorption time histories for any pixel can be taken out from the video frames. Finally, full data cubes can be measured, and each spectrum can be extracted for analysis.
Experimental Procedure
The Spero microscope’s standard configuration, with 12.5 x 0.7 NA objective, has a field-of-view of 650 µm x 650 µm and is used for imaging. The data cubes were gathered in transmission mode, across the range of 1800 to 900 cm- 1.
Using discrete frequency illumination at 1640, 1442 and 1200 cm-1, the video was recorded, which corresponded to the approximate absorbance maxima of H-O-H, H-O-D, and D-O-D respectively. The video was recorded in greyscale and a variety of high contrast color palettes, available within the ChemVision software.
The acquisition time was only 5 minutes, due to the recording of hyperspectral image cubes at a resolution of 4cm-1 across a spectral range of 1800 to 900cm-1. A 3 mm thick CaF2 window was used as a background reference for the images and data cube. A custom microfluidics cell with a “+” junction, offering configurations for laminar and shield flow, or up to three streams integrating at right angles was used. The channels were approximately 100 µm in width formed within a 20 µm thick PTFE spacer between two CaF2 windows.
Using a syringe pump, the flow was controlled; (New Era, model NE-400) fitted with 5 mL plastic barrel syringes. The data cube’s flow rate and corresponding videos were about 20 µL an hour, as reported by the syringe pump. Deuterium oxide, (Cambridge Isotope Laboratories Inc.) and deionized water were used at room temperature. The cell’s temperature was not controlled.
Instrument control, data cube, and video acquisition was made possible with the ChemVisionTM, Daylight Solutions software. MATLAB (MathWorks) and ImageLab (Epina GmbH) were used in post-processing.
Results and Discussion
Figure 1 illustrates the interaction of H2O with D2O at a flow rate of about 250 µL/minute as the cell was originally primed. Images were obtained at frequencies corresponding to the D-OD and H-OH absorbance maximums. Each image represents an area of 650 µm x 650 µm, and consists of 230,400 individual pixels.
.jpg)
Figure 1. Discrete frequency images of H2O and D2O flow within a microfluidic channel at characteristic absorbances. Video recorded at 1640 cm-1 displays the transmission image where H2O is black and D2O is white.
The ChemVision software images can be obtained in greyscale or numerous color schemes to improve contrast. Each pixel is a transmittance value, ratioed to the previously measured background. In these images, H2O flows into the cell in the two channels at a right angle to the intruding D2O stream. The image reveals the shape of the intrusion zone and the clear delineation of the two liquids. The PTFE cell spacer has an absorbance band coinciding with the D2O absorbance (greatest at 1200 cm) and therefore appears dark in the image.
The images in Figure 2 are still frames taken from the video clips recorded at the H2O, D2O and HOD absorbance maxima, with a flow rate of 20 µL/h.
At this flow rate, restriction prevented the flow of water from the lower channel. Illumination at 1640 cm-1 reveals a laminar flow at the interface of the H2O and D2O streams. Modifying the frequency to the absorbance maximum of HOD at 1442 cm-1, the area in which the proton/deuteron exchange is taking place and its distribution plume is obviously visible.
As in the earlier figure, a video at each frequency can be turned on using the mouse and left-clicking within the image. To demonstrate the dynamic nature of the flow, the pump was pulsed frequently which perturbed the flow to represent motion.
.jpg)
Figure 2. Discrete frequency video of H2O and D2O interface with proton deuteron exchange zone clearly observed at 1442 cm-1
Figures 1 and 2 were taken using Spero's real-time chemical imaging mode. It is obvious that spatial chemical data can be acquired by selecting the appropriate frequency for the functional group(s) of interest.
The next step is to gather the full hyperspectral image cube. In this mode, the entire IR frequencies are measured resulting in a 2D spatial image of the sample on the X-Y axes, and IR absorbance on the Z-axis. In this mode, it is possible to extract complete, fully ratioed IR spectra at any of the 230,400 pixels.
It is possible to obtain kinetic data from the experiment in multiple ways. Spectra taken from the image cube can be connected to the cursor position. With a click of the left mouse, the image on the page will animate the cursor and display the connected spectra. The color pallet utilized depends on the recorded absorbance intensity, ranges from low (blue) to high (red), and matches the HOD concentration.
The plume of HOD is obviously visible, as is the concentration gradient spanning the flow interface. The spectra from a line of pixels through this interface were extracted and applied to build plots of normalized net area versus pixel number, as illustrated in Figure 4. At this point, integrated area normalized to unity from the characteristic absorbance bands for H2O, D2O, and HOD has plotted versus pixel location.
This plot reveals the highest concentration of HOD is as expected for a 1:1 reactant to product ratio, to the point at which the H2O and D2O integrated net absorbance intersects. With calibration, this absorbance plot can be directly linked to concentration. Images can be shown at a number of frequencies using many contrast enhancements, such as bivariate or univariate peak height or area ratios, to advanced multivariate chemometric algorithms.
Figure 3 illustrates the cell at the IR frequency corresponding to the HOD species, 1442 cm- 1.
The spectrum in the panel on the right matches the cursor location on the image to the left panel. This spectrum can be related to time, using a distance to time calculation if the flow rate is precisely known. Discrete frequency imaging can be used as in Figure 2 where 30 fps video is recorded over the time period of the reaction. Each pixel in the video frame has a completely ratioed absorbance (or transmittance) value which can be extracted and plotted vs time calculated from frame number.
.jpg)
Figure 3. ImageLab display of the image cube of D2O/H2O flow showing absorbance image at 1442 cm-1 corresponding to HOD absorption. Red indicates high absorbance. Linked spectrum at right corresponds to the cursor position.
.jpg)
Figure 4. Histogram plot of normalized area vs. pixel for D2O, H2O, and d HOD
Spectral data in the image cube can be shown in many forms, from simple waterfall plots of spectrum vs pixel position (as in Figure 5) to intricate 3D illustrations of parts within the flow channel. Figure 6 displays the 3D illustrations of the concentration of H2O (blue), HOD (red) and D2O (green) within the fluidic channel.
.jpg)
Figure 5. Waterfall plot of H2O, D2O and HOD spectra across the H2O, D2O interface.
.jpg)
Figure 6. 3D representations based on the concentration of H2O (blue), HOD (red) and D2O (green) within the fluidic channel.
Summary
Infrared spectroscopy is an important tool that can identify, measure, and trend reaction components over time. Leveraging this capability Spero quantifies chemical content across large areas and visualizes their interaction and distribution in real-time.
Spero offers the novel ability to visualize parameters, such as the spatial distribution of reactants, products, and intermediates, flow dynamics diffusion/partitioning, advection and transport, concentration, and kinetics within microfluidic and flow chemistry experiments.
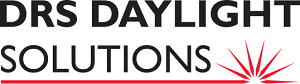
This information has been sourced, reviewed and adapted from materials provided by Daylight Solutions Inc.
For more information on this source, please visit Daylight Solutions Inc.